Canada’s Oceans Now: Case Studies from State of Canada’s Arctic Seas Report, 2019
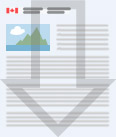
Canada’s oceans now: Case studies from state of Canada’s Arctic seas report, 2019
(PDF, 2.19 MB)
On this page
- Document information
- Box: Polar bears and the decline of sea ice
- Box: The ocean in bloom
- Box: Ringed seals and the decline of sea ice
- Box: A different year
- Box: Arctic sanctuary
- Box: Range expansions
- Box: Arctic hotspots
- Box: Community monitoring
- Box: Knowledge integration
- References
Document information
Editor. Andrea Niemi, Fisheries and Oceans Canada
Central and Arctic Region
501 University Crescent
Winnipeg, MB
R3T 2N6
Case Studies are taken from:
Niemi, A., Ferguson, S., Hedges, K., Melling, H., Michel, C., et al. 2019. State of Canada’s Arctic Seas. Can. Tech. Rep. Fish. Aquat. Sci. 3344: xv + 189 p.
© Her majesty the queen in right of Canada, as represented by the minister of Fisheries and Oceans Canada, 2019
Cat. No. Fs23-549/2-2019E-PDF
ISBN: 978-0-660-33144-7
Box: Polar bears and the decline of sea ice
An Apex Predator’s Response to Sea-ice Habitat Declines in Western Hudson Bay
Evan Richardson, Environment and Climate Change Canada
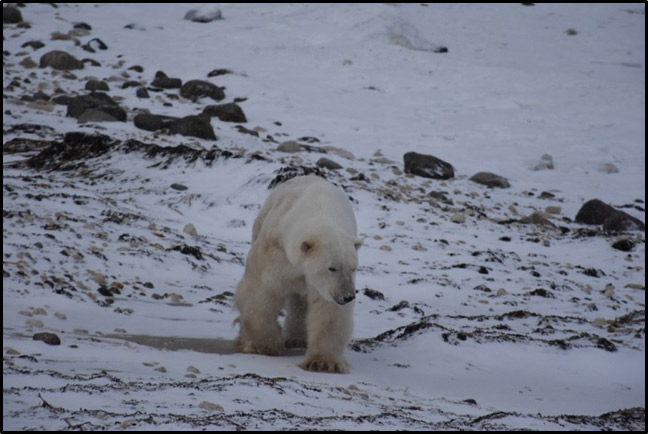
Figure 1. Polar bear on shoreline of Hudson Bay (photo credit: Evan Richardson).
At a global scale habitat loss represents one of the greatest threats to species conservation and in the Arctic long-term declines in the spatial and temporal extent of sea ice are predicted to have significant impacts on ice-obligate marine mammal species. Almost two decades ago research scientists began seeing the impacts of climate change in the Arctic’s most charismatic top predator, the polar bear (Ursus maritimus) (figure 1). At that time polar bears were beginning to lose body condition in western Hudson Bay in relation to reductions in sea-ice extent and the availability of their primary prey, ice-breeding seals (Stirling et al. 1999). Similar trends in the body condition of ringed seals (Pusa hispida) in relation to sea-ice extent have now been observed in Hudson Bay (Ferguson et al. 2017) indicating that sea ice may not only be influencing the availability of prey but also the quality of prey being consumed. These important interspecific interactions have likely played an important role in the continued, observed long-term declines in polar bear body condition at the southern limit of their range (Obbard et al. 2016; Sciullo et al. 2016).
Polar bear life history is intimately linked to the sea ice which provides a platform on which polar bear hunt, travel, mate and den. During the spring period polar bears prey heavily upon young ringed seal pups that are naïve and provide a high caloric food source. However, recent evidence suggests that ovulation and thus ringed seal pupping rates may be influenced by long-term variations in the Arctic marine environment (Ferguson et al. 2017), further bringing into question the role sea ice plays in the interactions between these two species.
As a result of reductions in sea-ice extent (Figure 2), polar bears are now spending more time on land and have begun to make use of alternative food resources. However, polar bear life history and physiology have evolved to make use of lipid rich marine mammal prey, thus terrestrial food resources, such as sea bird eggs, are not expected to maintain polar bear body condition in the face of long-term reductions in sea-ice extent (e.g., seabirds). There is some evidence to suggest that similar to ringed seals, polar bear are possibly becoming more stressed in relation to long-term reduction in the availability of prey (ECCC, unpublished data). Reductions in sea-ice extent are also predicted to increase the number of human-polar bear interactions (Towns et al. 2009), potentially influencing individual survival. What does all this mean for polar bear populations?
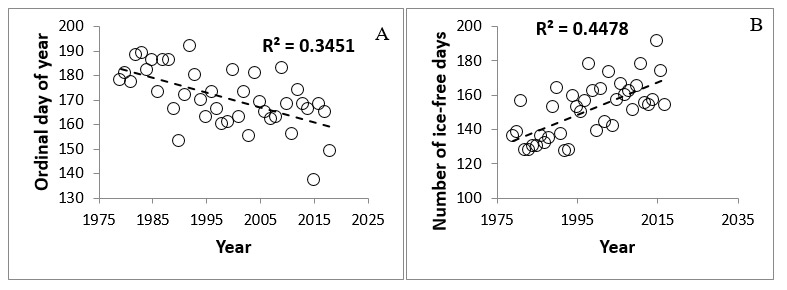
Figure 2. (a) Long-term declines in the date of sea-ice break-up and (b) increases in the duration of the ice-free period in western Hudson Bay Canada (data source: National Snow and Ice Data Center, https://nsidc.org/data/NSIDC-0192).
Long description
Figure 2. Plot A. A scatter plot of the long-term decline in the date of sea-ice break-up in western Hudson Bay. The decline has an R2 value of 0.3451. X-axis (bottom) is years from 1975 to 2017. Y-axis (side) is the Ordinal day of the year from 130 to 200. Plot B. A scatter plot of the long-term increase in the duration of the ice-free period in western Hudson Bay Canada. The increase has an R2 value of 0.4478. X-axis (bottom) is years from 1975 to 2017. Y-axis (side) is the number of ice-free days from 120 to 200.
In 2016 Lunn et al. published a long-term analysis of polar bear population demography in western Hudson Bay examining individual variation in survival in relation to changes in sea-ice dynamics. They found that survival of both young and adult female polar bears was related to changes in sea-ice dynamics that influenced the availability and quality of polar bear habitat. At the same time they were able to document a 30% decline in the size of the western Hudson Bay polar bear population from 1987-2011 (Figure 3). Subsequent aerial surveys have confirmed the population has declined to approximately 842 bears (Dyck et al. 2017).
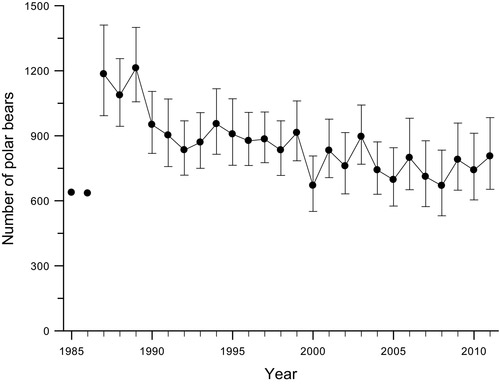
Figure 3. Long-term declines in the size of the western Hudson Bay polar bear population from 1987-2011 mediated by reductions in the availability of sea ice (source: Lunn et al. 2016).
Long description
A line graph of long-term declines in the size of the western Hudson Bay polar bear population from 1987-2011 mediated by reductions in the availability of sea ice (source: Lunn et al. 2016). The x-axis (bottom) is years (1987 to 2011) and the y-axis (side) is number of polar bears with data spanning between ca. 600 and 1400. After 1986, each data point has an error bar associated with it. The error bars show a plus/minus of 10 to 15%.
The mechanistic relationship between sea-ice dynamics, polar bear body condition, and ultimately polar survival have now been observed elsewhere. Similar declining trends in body condition initially observed by Stirling et al. (1999) in western Hudson Bay have now been documented in southern Hudson Bay (Obbard et al. 2016). More recently an aerial survey has shown a ~ 17% decline in the southern Hudson Bay polar bear population from 2011-2016 (Obbard et al. 2018). From an energetics perspective these data collectively highlight the importance of sea ice to polar bear foraging ecology and the downstream demographic responses that can result from long-term declines in body condition. Similar responses in prey populations (e.g., declines in ringed seal body condition leading to reduced survival) could have cascading effects on the long-term persistence of polar bears across their range.
Box: The ocean in bloom
Changing Phytoplankton Phenology: From Shelves to Basins
Pierre Coupel, Christine Michel, Emmanuel Devred, Fisheries and Oceans Canada
The timing of events in the annual cycle (i.e., phenology) affects the functioning of the entire marine ecosystem. The spring to early summer phytoplankton bloom is often considered as the single most important event in the seasonal cycle of production in the Arctic, aside from the bloom of ice algae. The transfer of primary production from the short-lived phytoplankton bloom to upper trophic levels depends not only on the temporal and spatial coupling between grazers and the timing of bloom occurrence, but also on the taxonomic composition of the bloom. In the warming Arctic, earlier sea-ice retreat and later freeze-up are changing the phenology, of the phytoplankton bloom. Predictions for a second fall bloom due to longer open-water seasons (Kovacs and Michel 2011) are now documented throughout the Arctic and on Canadian shelves (Ardyna et al. 2014; Michel et al. 2015).
The loss of thick multiyear ice and the overall thinning of Arctic sea ice is also cause for a marked increase in the prevalence of light conditions conducive to under-ice blooms. Over the past decades, earlier and extensive sea-ice melt has resulted in conditions suitable for the development of under-ice blooms, such that nearly 30% of the ice-covered Arctic Ocean has become favourable for under-ice phytoplankton blooms in July (Horvat et al. 2017). One of the important consequences of under-ice blooms is that they consume a substantial fraction of surface nutrients at the expense of primary production in the marginal ice zone following ice retreat (Palmer et al. 2014). While both ultraviolet radiation and zooplankton grazers have little effect on under-ice primary production, they can reduce the magnitude of primary production in open waters, with important implications for the production that is transferred to pelagic or benthic food webs. The shift in the timing of primary production can also impact total ecosystem productivity by changing the balance between production occurring within and under the ice and that in open waters, and affect food-web transfers through a mismatch with grazer life cycles that can have cascading implications on higher trophic levels.
Superimposed on these changes in seasonality, one also observes a shelf-to-basin displacement of the ice edge. Because the location of the ice edge relative to topography is a key parameter for upwelling and mixing (Carmack and Chapman 2003), knowledge of the spatio-temporal distribution of the ice edge is crucial to understand and predict changes in the magnitude and type of primary producers and the food webs that depend on them in the changing Arctic. A schematic of changes in the phenology of primary production on the shelves and basins, due to climate warming, is presented in Figure 4 and described below.
On productive Arctic shelves, the spring ice break-up typically triggers a short and intense ice edge bloom, dominated by large diatoms such as Chaetoceros spp. and Thalassiosira spp. These large phytoplankton store energy in lipid form and are very efficient at transferring energy to harvestable resources, compared to smaller phytoplankton. In areas still ice-covered, ice algal or under-ice phytoplankton blooms can develop when sufficient light is transmitted through the ice cover. Following these events, the nature of a summer phytoplankton bloom changes drastically from that of an ice-edge bloom. In response to nutrient depletion at surface, the bloom reaches deeper in the water column where it forms a so-called sub-surface chlorophyll maximum (SCM). As nutrients become depleted, small phytoplankton cells replace the diatoms and low light-adapted species such as Micromonas sp. take precedence as solar radiation declines and winter sets in.
In recent years, ice edge blooms have developed offshore. Although dominated by diatoms, the offshore blooms are one order of magnitude less productive than blooms occurring on the shelf, owing to the lower initial surface nutrient inventory in the basin (Coupel et al. 2015). Concomitantly, on the shelf, the location and timing of productive hot spots is likely to change since it depends on the balance between mixing that provides nutrients and light availability, both linked to the location of the ice edge. At the shelf break, upwelling-favourable wind conditions can generate productive fall blooms depending on ice conditions and available daylight.
Regional differences in the timing and extent of the open-water surface area have important implications for phytoplankton bloom phenology (Barber et al. 2015). Phytoplankton blooms in the Atlantic-influenced Barents Sea start as early as late April, whereas in the Canadian sector, phytoplankton blooms usually develop after mid-June (Mundy et al. 2014) or mid-July (Arrigo et al. 2012). Overall, complex sea-ice dynamics influence the timing, location, magnitude and composition of primary producers on Arctic shelves and deep basins. Our overall understanding of phytoplankton phenology in relation to ice dynamics is being challenged by the rapid on-going changes, and the effects of the trophic cascade remain to be understood.
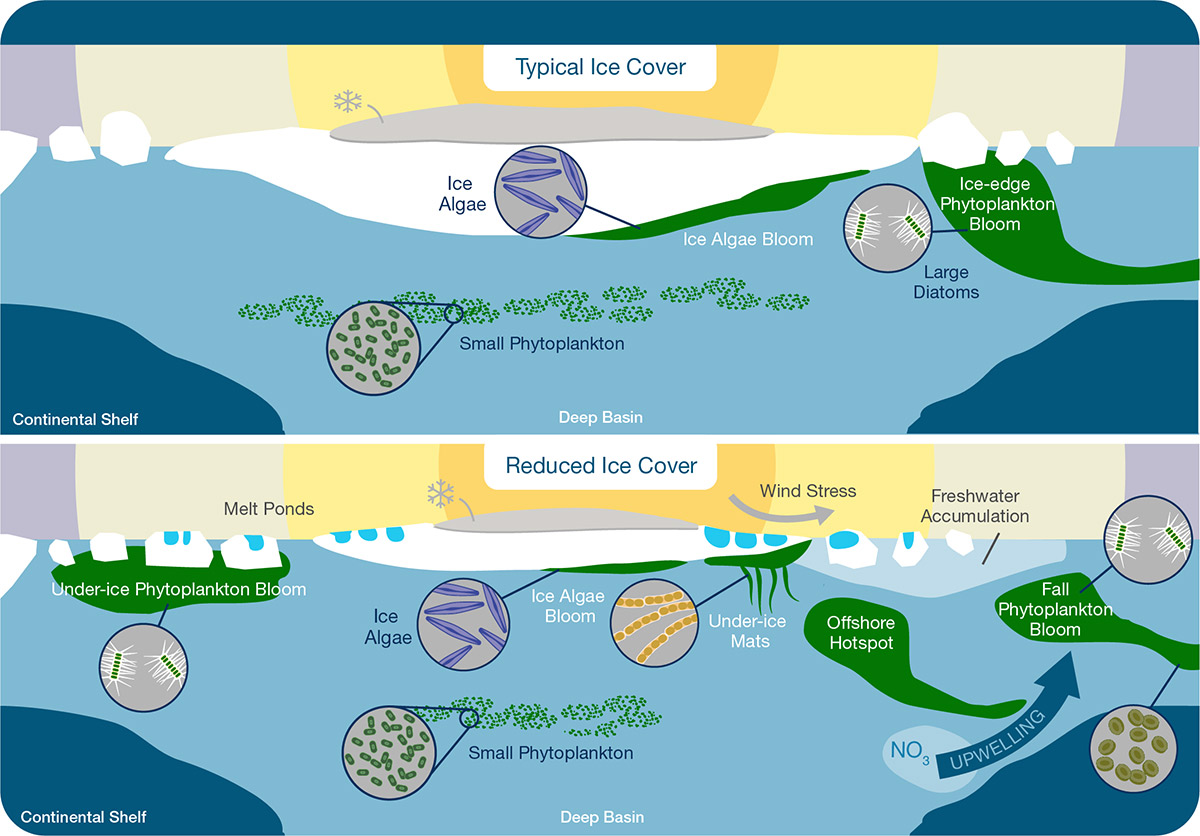
Figure 4. Annual events (phenological changes) in sea-ice and water column primary production under typical (top panel) and reduced (bottom panel) ice-cover conditions on Arctic shelves and basins.
Long description
An infographic (cartoon) depicting annual events (phenological changes) in sea-ice and water column primary production under typical (top panel) and reduced (bottom panel) ice-cover conditions on Arctic shelves and basins.
In the top panel (typical ice cover scenario), the sea ice is thicker and breaks up in the late spring/summer period. There is more snow on the sea ice. In the spring, ice algae grow at the bottom of the sea ice. The ice algae are primarily comprised of pennate diatom species. When the ice breaks up in spring/summer there is a short and intense ice-edge phytoplankton bloom dominated by different species of large diatoms. Under typical conditions, this ice-edge bloom generally occurs when the ice is located over the continental shelf. In the summer, the deep water area has a bloom comprised of small phytoplankton species.
In the bottom panel (reduced ice cover scenario), the sea ice is thinner with less snow on top. The ice is breaking up earlier in the season and the spring/summer ice has areas of melted snow and ice, called melt ponds, on its surface. There is a layer of accumulated freshwater at the surface during the ice melt period. Ice algae still grow at the bottom of the ice in spring/summer and over deep basin water, different species of ice algae may grow creating under-ice mats that hang down in long strands. An under-ice phytoplankton bloom develops and it is smaller than the ice-edge phytoplankton bloom under typical conditions. The bloom of small phytoplankton in basin waters is also smaller than under typical ice conditions. Under the reduced ice scenario there may also be an offshore hotspot bloom of phytoplankton and in the fall winds may mix the water creating upwelling that carries nitrate (NO3) to surface waters where a fall-time phytoplankton-bloom can develop that changes from large diatom to small diatoms over time.
Box: Ringed seals and the decline of sea ice
An Extreme Warm Event in Hudson Bay and Ringed Seal Response
Steve Ferguson, Fisheries and Oceans Canada.
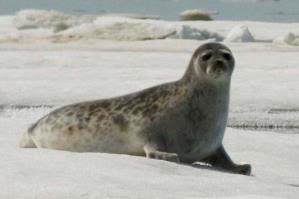
Figure 5. Ringed Seal on sea ice (source: NOAA Seal Survey, public domain, via Wikimedia Commons).
One of the main story lines of climate change is the loss of sea ice in Polar Regions, particularly as it relates to critical habitat loss for mammals. Over the last 2-3 decades, marine mammals studied in the Beaufort Sea have responded differently to changes in the extent and persistence of sea ice. The condition of young bowhead whales (Balaena mysticetus) improved over time, whereas ringed seals (Pusa hispida) and beluga (Delphinapterus leucas) experienced declines in condition (Harwood et al. 2015a). Questions remain, however, with respect to the actual causes of these divergent responses and what such responses mean for a given species or marine ecosystem. A recent study in the Canadian Arctic focused on demographic changes (e.g., disruption to reproduction, low pup survival, high mortality) of ringed seal (Figure 5) populations related to environmental stressors.
Seasonality of sea ice is critical for ringed seals. They require sea ice in the spring when they molt, an extended time period during which they shed their fur and skin and grow a new coat, and reproduce. In the ice-free summer season, ringed seals forage in open water and build up their fat stores. During winter, they give birth and nurse but are restricted to smaller ranges. While they are well-adapted to the seasonality of sea ice, sea ice is declining in response to warming. Ferguson et al. (2017) examined ringed seals in Hudson Bay, one of the southernmost reaches of their distribution, as well as the extent of sea ice. The area goes through a complete cycle of ice loss and re-formation (Figure 6).
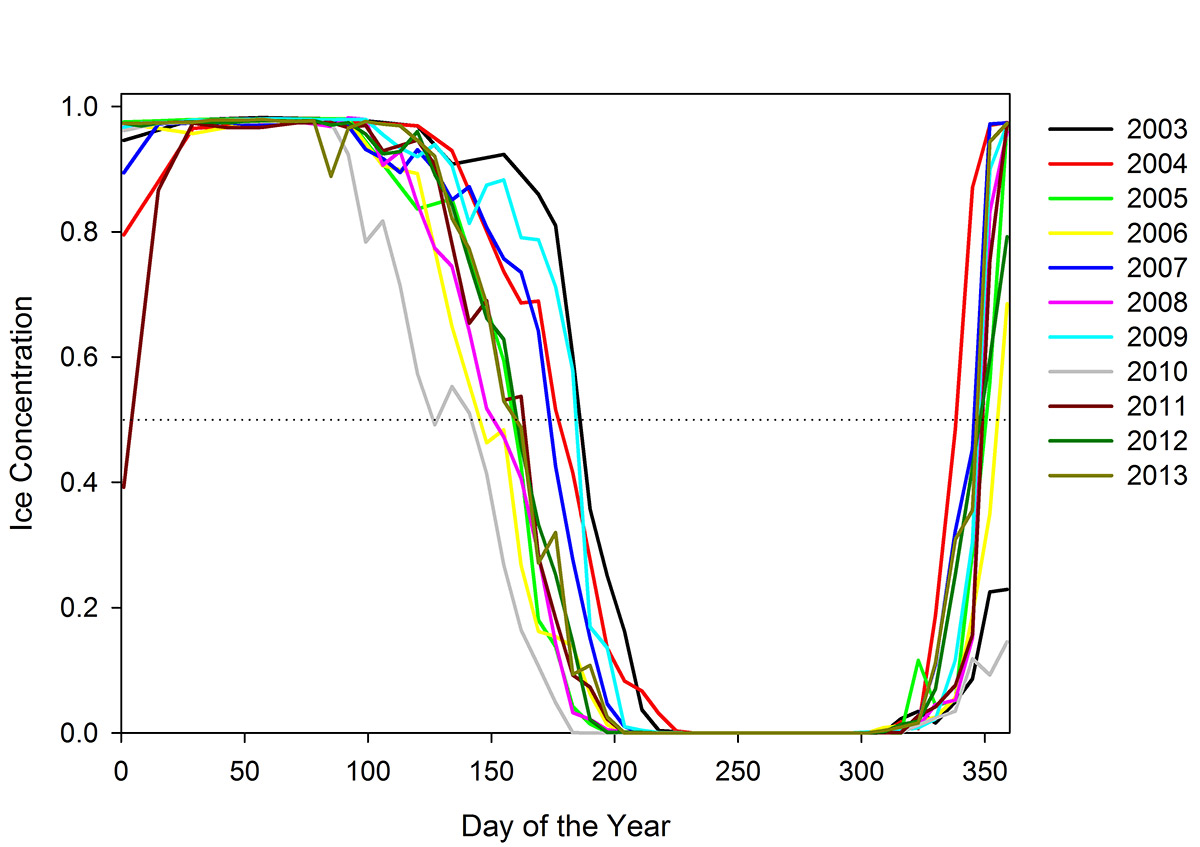
Figure 6. Sea-ice concentration each day of the year from 2003-2013 in Hudson Bay. The various colours represent different years. 50% concentration is shown by the dotted horizontal line and is considered the breakpoint for sea-ice break-up (<50%) and freeze-up (>50%). Note grey (2010 lowest concentration to left) and purple (2011 lowest concentration to right) years (source: Ferguson et al. 2017).
Long description
Line graph of sea-ice concentration each day of the year from 2003-2013 in Hudson Bay. Each year is represented by a different color line. The x-axis (bottom) is day of the year from 0 to 365. The y-axis (side) is ice concentration on a scale of 0 to 1. There is a dotted horizontal line at 0.5 or 50% ice concentration and is considered the breakpoint for sea-ice break-up (<50%) and freeze-up (>50%). All lines approach 100% ice concentration around day 50 and then decline to zero over a period of ca. 100 days (e.g., day 125 to day 225) illustrating that Hudson Bay is ice free in the summer. Lines increase after day 300. Of note is the grey 2010 line that is lowest on the left hand side of the graph (i.e. early break-up) and the purple 2011 line that shows lowest ice concentration on the right side of the graph.
By analyzing sea ice and climate data it was evident that sea ice is gradually breaking up earlier and freezing up later in Hudson Bay, indicating that the ice season is getting shorter. Between 1979 and 2014, there was no relationship between any of the climate indices and the dates of break-up or freeze-up. What this means is that the shortening of the sea-ice season is not related to natural climate patterns, but instead likely a direct result of human-induced climate change. The longest ice-free season occurred in 2010 with ice break-up arriving in May and not freezing again until January of 2011 (Figure 6).
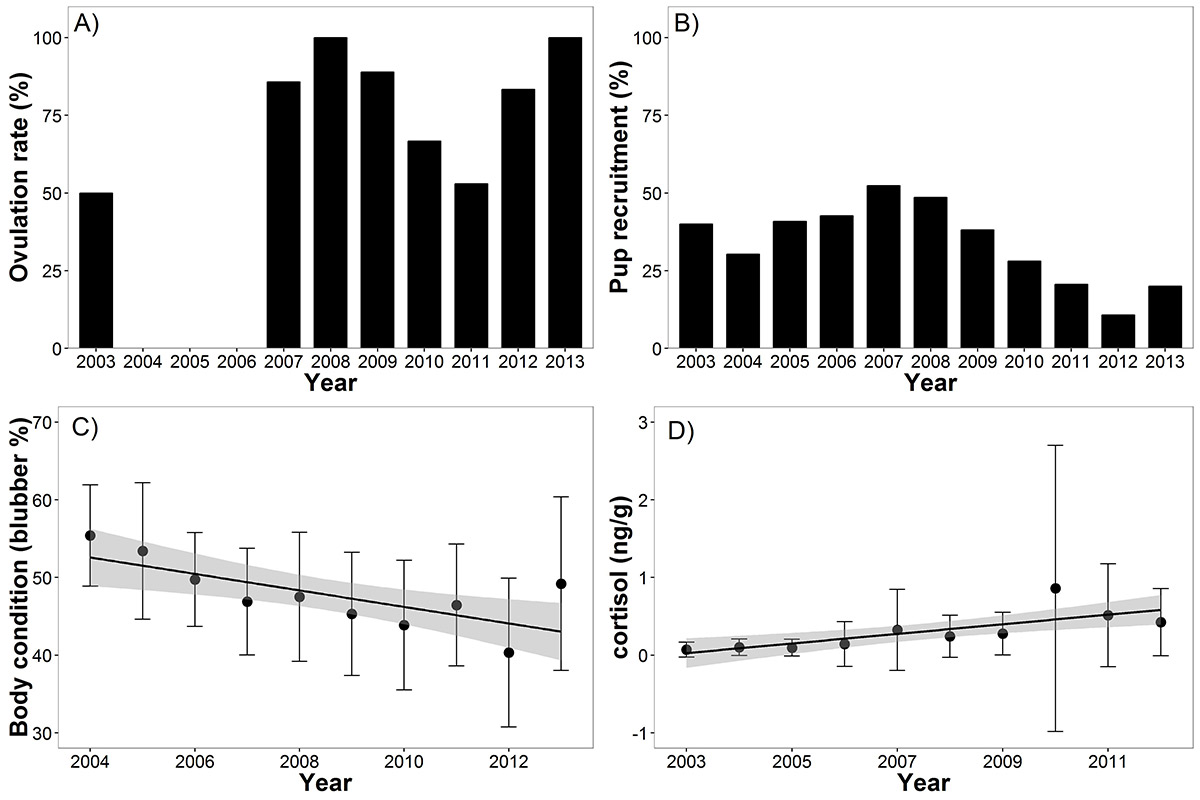
Figure 7. a) Annual ovulation rate each year (note 2011 low), b) Annual percentage of pups in the harvest (a representation of pup recruitment; note recent declining trend), c) relationship between seal body condition and year (note decline), d) relationship between cortisol (stress) level and year (note increase over time) (source: Ferguson et al. 2017).
Long description
The figure is comprised of 4 graphs pertaining to ringed seals in Hudson Bay. Graph A (bar graph) shows the percent ovulation rate (y-axis) for female ringed seals for years 2003 to 2013. Of note to the discussion is a low ovulation year, only slightly above 50%, in 2011. The rates for 2007 to 2013 are variable and the majority of bars are between 75 and 100%. Data is not available for 2004-2006. Graph B (bar graph) shows the percentage of ringed seal pups in the community harvest from 2003 to 2013. Pups in the harvest is used as a representation of pup recruitment (survival of one life stage to the next). A declining trend is noted from 2007 to 2013. Graph C (line graph) shows the relationship between ringed seal body condition defined as percent blubber on the y-axis between 2004 and 2014 on the y-axis. Each data point has an error bar associated with it and there is an overall decline in condition. Graph D (line graph) shows the relationship between ringed seal stress level measured as cortisol concentrations in nanogram per gram, for the years 2003 to 2012. Each data point has an error bar associated with it and there is an overall increase in stress over time.
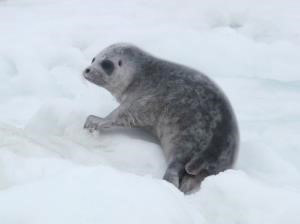
Figure 8. Ringed seal pup (source: Shawn Dahle, NOAA, Polar Ecosystems Program research cruise, public domain, via Wikimedia Commons).
By examining body condition, reproductive condition, pup recruitment, and stress level from 1425 seals harvested as part of Inuit subsistence hunting in Hudson Bay between 2003 and 2013 it was found that ringed seal body condition declined from 55% blubber mass in 2004 to only 40% in 2012, with the caveat that it did increase again to 48% in 2013. The decline in body condition was related to the increased period of open water (shorter sea-ice season). Cortisol concentration (a measure of stress) increased over time in ringed seals. In 2010, cortisol levels were high and showed high variability. Ovulation rates were low the following year (2011), likely attributable to the high stress ringed seals experienced in 2010 (Figure 7).
What might this mean and the bigger picture? This study showed declining ringed seal body condition is concurrent with sea-ice decline, one of the many consequences of climate change. In addition, the study documents a relationship between the 2010 climatic event and ringed seal demographic changes, as body conditions were reduced, seals were stressed, and ovulation in seals decreased, leading to fewer pups (Figure 8) in the following years. This climatic event is linked to large-scale climatic patterns indicating that climate-controlled cycles will continue to impact body condition and seal demographics. In Hudson Bay, seal condition showed some recovery in the years following 2010. However, the 2010 cohort will be few in number due to the extreme conditions of 2010.
What is the mechanism by which ringed seal body condition was reduced and stress increased by sea-ice loss (or other factors)? The longer open-water period of 2010 may have affected the ringed seals’ access to prey items as well as the abundance and distribution of the prey. Higher sea temperatures late into the fall may have resulted in hyperthermia in fat seals and seals hauling out on shorelines. The seals also showed evidence of anomalous lethargic behaviour which may indicate disease/illness, possibly a consequence of disrupted molting. Illness may have in turn increased their risk of predation by polar bears (Ursus maritimus) (Figure 9). Therefore, the mechanism behind declining seal body condition with longer open-water periods is not yet well understood.
According to long-term atmospheric patterns, episodic events such as the one in 2010 are expected to occur every 10-15 years but be unpredictable. It is the combination of climate-change-driven gradual sea-ice loss and these unpredictable episodic events that is most likely to have major implications for ringed seal body condition and over the long-term their abundance and distribution.
This research provides more information on what may be expected in the future, but prediction of the future is still elusive. Further research will be required to determine the mechanism behind body condition declines with sea-ice loss, how seal populations will adapt to climate change, and how the larger ecosystems will also be affected.
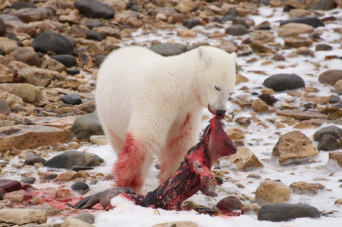
Figure 9. Polar bear eating a seal caught on land north of Churchill in October 2010 during the unusual year when ringed seals crawled out of the water in the vicinity of polar bears awaiting the return of sea ice (photo credit: Daryl Hedman).
Long description
Photograph of a polar bear on a rocky shoreline near Churchill Manitoba. The front legs of the polar bear are blood stained and it holds remains of its preferred prey, a ringed seal, in its mouth. The bear caught the seal on land during the low sea ice year of 2010 when seals crawled out of the water in the vicinity of polar bears to await the return of sea ice.
Box: A different year
Ecosystem Variability in the Southern Canadian Beaufort Sea
Andrew Majewski, Andrea Niemi, Jane Eert, Christine Michel, Ellen Lea, Lisa Loseto, Jim Reist, Fisheries and Oceans Canada
Maxime Geoffroy, Memorial University
In the past 15 years, considerable research attention has focused on the Beaufort Sea marine ecosystem. Federally administered research including the Northern Coastal Marine Studies program (DFO, 2003-2009), ArcticNet (2003-), the Beaufort Regional Environmental Assessment (BREA, 2011-2015), and the current Canadian Beaufort Sea – Marine Ecosystem Assessment (CBS-MEA, 2017-) have established biological baselines for the offshore marine environment and, for the first time, are beginning to build time series of biological data associated with both physical and chemical habitat characteristics. Results indicate extensive spatial and interannual variability for several key ecosystem components, presenting the challenge of identifying natural variability in an environment that is also rapidly changing in relation to climate change. Such challenge also applies to other regions of the Canadian Arctic, many of which are poorly studied.
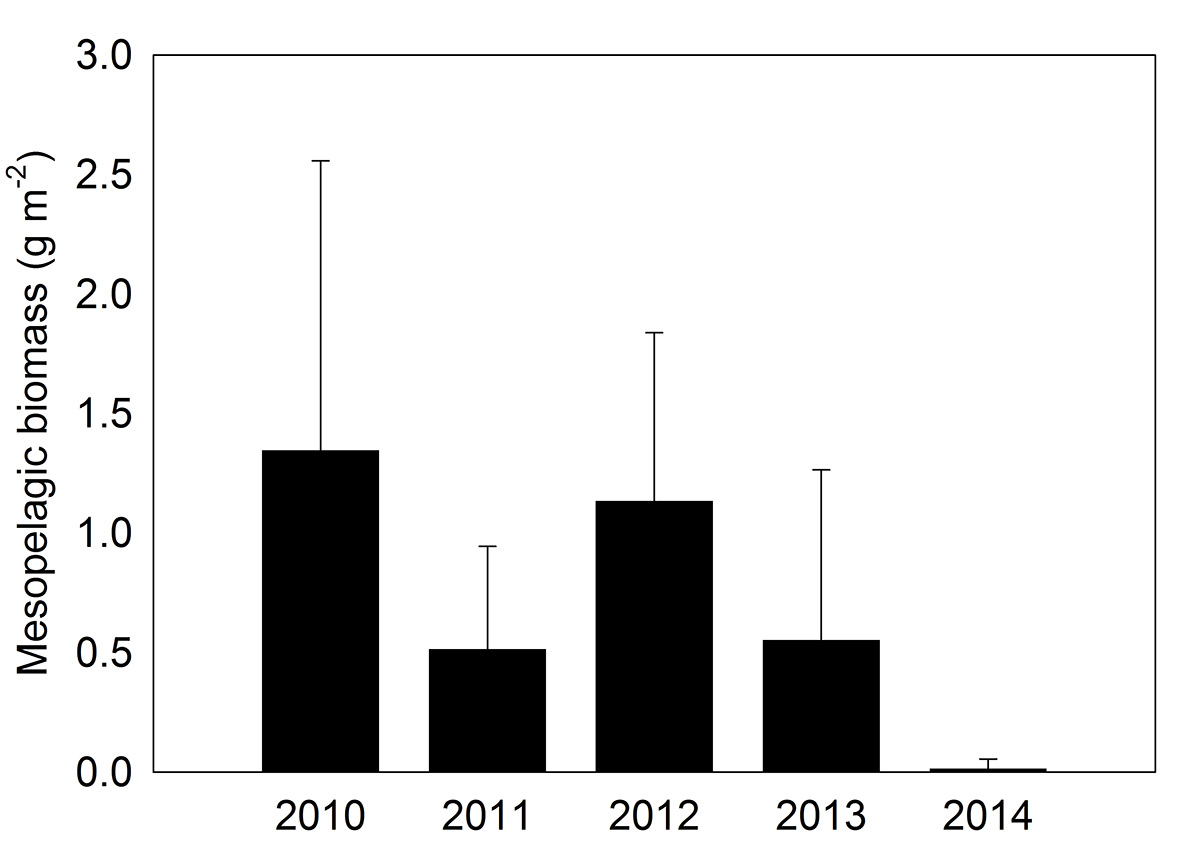
Figure 10. Mesopelagic biomass of Arctic Cod in the southern Canadian Beaufort Sea, calculated from hydroacoustics data (source: M. Geoffroy, unpublished data).
Long description
Bar graph of Arctic Cod mesopelagic biomass on the y-axis (side), ranging from 0 to 2.5 grams per meter squared, and years 2010 to 2014 on the x-axis (bottom). Each bar has an error bar associated with it. The biomass values have been calculated from hydroacoustics data. The data shows high variability between the years with a very low biomass year, less than 0.1 grams per meter squared, in 2014. 2010 and 2012 have mean biomass values between 1 and 1.5 grams per meter squared and 2011 and 2013 have mean biomass values between 0.5 and 0.75 grams per meter squared.
Arctic Cod (Boreogadus saida) are an important component of the Beaufort Sea marine forage base, and play important roles both as grazers and as prey for fish, seals, whales and birds (Mueter et al. 2016). Joint hydroacoustic surveys conducted aboard the CCGS Amundsen and F/V Frosti between 2010 and 2014 indicated substantial interannual variability in the biomass of Arctic Cod in both young-of-year (age-0) and adult life-stages, with a sharp decline in biomass of adult cod observed in the 2014 sampling year (Figure 1 0). This decline was preceded by a general decline in the abundance and biomass of age-0 cod between 2010 and 2013. A subsequent analysis incorporated these data along with ship-based acoustic data sets from across the Canadian Arctic and determined that the biomass of age-0 cod in August and September was negatively correlated to ice breakup week and positively correlated to sea-surface temperature (Bouchard et al. 2017), suggesting bottom-up control for some trophic levels, such as pelagic fish.
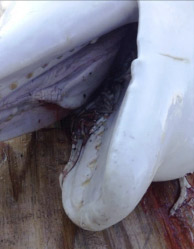
Figure 11. Beluga whale with mouthful of Sand lance (photo courtesy of Loseto et al. 2018a).
Concurrent with the low biomass of adult Arctic Cod in the Canadian Beaufort in 2014, remarkable observations occurred in other aspects of the ecosystem, including coastal areas. Over 30 beluga whales were harvested near Ulukhaktok, which was the only harvest of this magnitude on record for that community (Loseto et al. 2018a). Observations from monitoring programs in the Mackenzie Estuary and Darnley Bay indicate that beluga stomachs are typically empty upon harvest (Harwood et al. 2015b). The diet of Eastern Beaufort Sea belugas has mainly been inferred from biotracer studies (Loseto et al. 2009), investigations of habitat use during tagging studies (Hauser et al. 2017), and aerial surveys (Hornby et al. 2016, 2017). These studies all pointed to Arctic Cod as a primary prey species for beluga in offshore marine waters. The belugas harvested at Ulukhaktok in 2014 were unique in having numerous prey items in their stomachs, but did not appear to be feeding substantially upon Arctic Cod. Instead, Sand lance (Ammodytes spp.) (Figure 1 1) was the numerically dominant prey item in guts, along with squid (inferred by beaks) and other fish species to much lesser extent (Loseto et al. 2018a). Beluga harvested in the Inuvialuit Settlement Region in 2014 had lower body condition index values than those harvested the previous three seasons, suggesting that annual variability in prey may be associated with inter-annual variation in condition of beluga whales (Choy et al. 2017).
Gut contents of Arctic Char (Salvelinus alpinus) harvested in Ulukhaktok in 2014 and 2015 indicated that their diet can be highly variable, and may be linked to the availability of preferred prey. As with beluga, Sand lance was prevalent in the diet of Arctic Char, as were marine amphipods. These results contrast with samples collected in Ulukhaktok in 1977 and 1978 that contained predominantly Arctic Cod (E. Lea, unpublished data).
Apparent inter-annual variability in the Beaufort Sea ecosystem was also indicated by primary production estimates. Despite an apparent low biomass year for Arctic Cod in 2014, and concurrent shifts in distribution and diet of subsistence species, the biomass of primary producers, indicated by chlorophyll a concentrations, reached values six times higher than in 2013 or 2017, with highest biomass accumulation in the southwest Banks Island area (C. Michel, unpublished data) (Figure 12). These results suggest that ample resources were available to support strong secondary productivity, at least at localized scales.
Results and observations from the open-water season in 2014 provide a distinct example of interannual variability that occurred at multiple trophic levels, and was observed at both offshore and coastal environments. Variability affects subsistence harvests within the area, creating benefits and uncertainties for different communities (Loseto et al. 2018a). Given the limited number of years of sustained, ecosystem-level observations in the Canadian Beaufort Sea, it is not possible to label 2014 as an ecological anomaly. Further work is required to understand the drivers of the observed ecosystem changes, acknowledging that the drivers may have initiated the changes in seasons or years prior to the 2014 open-water season. It is expected that variability and the occurrence of anomalous events will likely become more common as the earth warms (IPCC 2014). Consequently, ecosystem monitoring required to understand the drivers of ecosystem variability, including physical and biological couplings, is essential to predicting local and down-stream effects of climate change in the Beaufort Sea, and for informing adaptation strategies in the Inuvialuit Settlement Region.
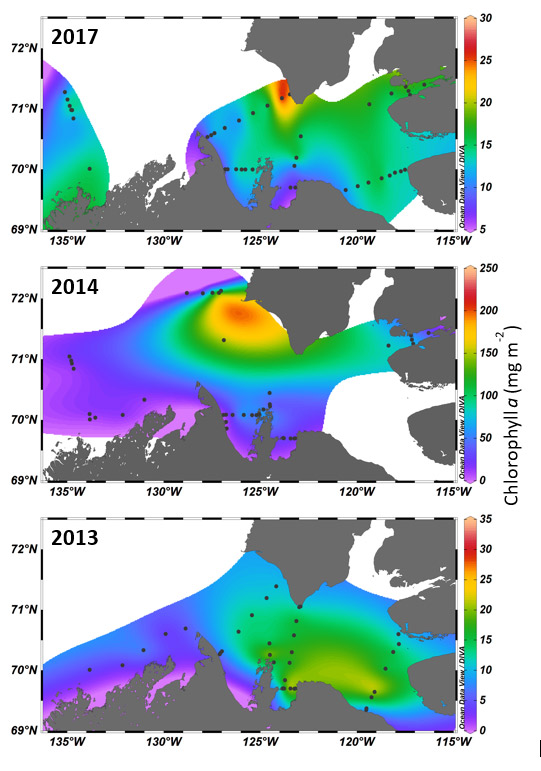
Figure 12. Beaufort Sea chlorophyll a concentrations, integrated for the euphotic zone, in 2013, 2014 and 2017. (source: C. Michel, unpublished data, Maps created with Ocean Data View, Schlitzer, R., https://odv.awi.de, 2018.).
Long description
Three map plots are shown that include the marine areas of the Beaufort Sea (offshore), the Mackenzie Shelf and Amundsen Gulf. The mainland of the Northwest Territories is along the bottom of each map and the southern portion of Banks Island and neighbouring eastern side of Victoria Island is along the top and right-hand side. Sampling station locations are plotted as black dots for the years 2017 (top), 2014 (middle) and 2013 (bottom). At each station, chlorophyll a concentrations were measured and integrated for the euphotic zone. The integrated chlorophyll a concentrations are presented as a surface color plot. Red and yellow areas indicate highest concentrations whereas purple and blue tones represent low concentrations. The integrated chlorophyll a concentrations, in mg per meter squared, ranged from near 0 to ca. 25, 200, and 30, in 2013, 2014 and 2017, respectively. 2014 chlorophyll reached values six times higher than in 2013 or 2017. Concentrations in all 3 years were highest in Amundsen Gulf with highest biomass accumulation in the southwest Banks Island area in 2014 and 2017.
Box: Arctic sanctuary
The North Water – Sanctuary for High Productivity and Associated Biodiversity
Cortney Watt and Christine Michel, Fisheries and Oceans Canada
During the ice-covered period, polynyas provide reliable areas of open water where marine mammals and birds congregate. The North Water in northern Baffin Bay between Greenland and Canada is the largest and best characterized polynya in the Canadian Arctic. The polynya owes its existence to an ice bridge typically forming in Nares Strait, which retains ice drifting from the Arctic Ocean, and to a combination of latent (wind-driven) and sensible (ocean-warming) heat processes.
Prior to the rapid Arctic sea-ice changes of the past two decades, the North Water, also known as Pikialasorsuaq, was considered one of the most productive regions of the Arctic (Deming et al. 2002). Pikialasorsuaq supports large populations of seabirds and marine mammals (Heide-Jørgensen et al. 2013), and human settlement on adjacent coasts dates back to 4,500 years BP (Jeppesen et al. 2018) demonstrating the importance and reliability of this area. There are 14 species of seabirds that regularly use the polynya for breeding and the most abundant is the little auk (Alle alle), with more than 30 million breeding pairs (Egeyang et al. 2003; Davidson et al. 2018). Breeding colonies of hundreds of thousands of thick-billed murres (Uria lomvia) and tens of thousands common eider (Somateria molissima) are also found in the region (Burnhan et al. 2012; Merkel et al. 2014). Marine mammals also depend on this polynya as a refuge from ice cover and access to air. Narwhals (Monodon monoceros), belugas (Delphinapterus leucas) and bowhead whales (Balaena mysticetus) are species that regularly frequent polynyas and leads during the winter period. In 2009 and 2010 it was estimated that over 27,000 marine mammals inhabited the North Water in the month of May, including beluga whales, narwhal, walrus (Odobenus rosmarus), ringed seal (Pusa hispida), bearded seal (Erignathus barbatus), and polar bears (Ursus maritimus) (Heide-Jørgensen et al. 2013). In April 2014, over 13,500 walrus, beluga, narwhal and bearded seals were seen in the region (Heide-Jørgensen et al. 2016). Marine mammals and seabirds also depend on other polynyas in the Canadian Arctic in southern Baffin Bay, Hudson Strait and Cumberland Sound (Lewis et al. 2009; Watt et al. 2016, 2017; Chambault et al. 2018).
The early opening of the North Water allows for the development of a phytoplankton bloom several months earlier than in nearby ice-covered waters of the Canadian Archipelago (Tremblay et al. 2006a; Michel et al. 2015). Efficient transfers to the pelagic ecosystem (Tremblay et al. 2006b) support the productive marine food web. A recent time-series analysis of remote sensing estimates of phytoplankton biomass in the North Water over the past two decades (1998 to 2014) shows a significant decline in the amplitude of the bloom, despite interannual variability in the observational series (Marchese et al. 2017). The high interannual variability is attributed to a fine balance between oceanographic and climatic forcings, with longer and shorter blooms during years of low and high ice cover, respectively. The recent decline in phytoplankton biomass and production is also documented by in situ measurements (Blais et al. 2017) (Figure 13), attributed to changing sea-ice conditions and delayed formation or absence of the ice bridge in Nares Strait.
Collectively, these results indicate that Pikialasorsuaq, a region long known for its recurrent and predictable high productivity and abundance of marine resources, has been negatively impacted by climate change over the past decade. In preparation for future changes, the Pikialasorsuaq Commission (pikialasorsuaq.org) is coordinating efforts in Nunavut and Northern Greenland to safeguarding, monitoring and management of the health of Pikialasorsuaq for future generations. In 2017 the Commission requested the creation of an Inuit-identified, Inuit-managed protected area in the ecologically and culturally significant area of the North Water (Pikialasorsuaq Commission 2017). An implementation framework for management options was developed in 2018.
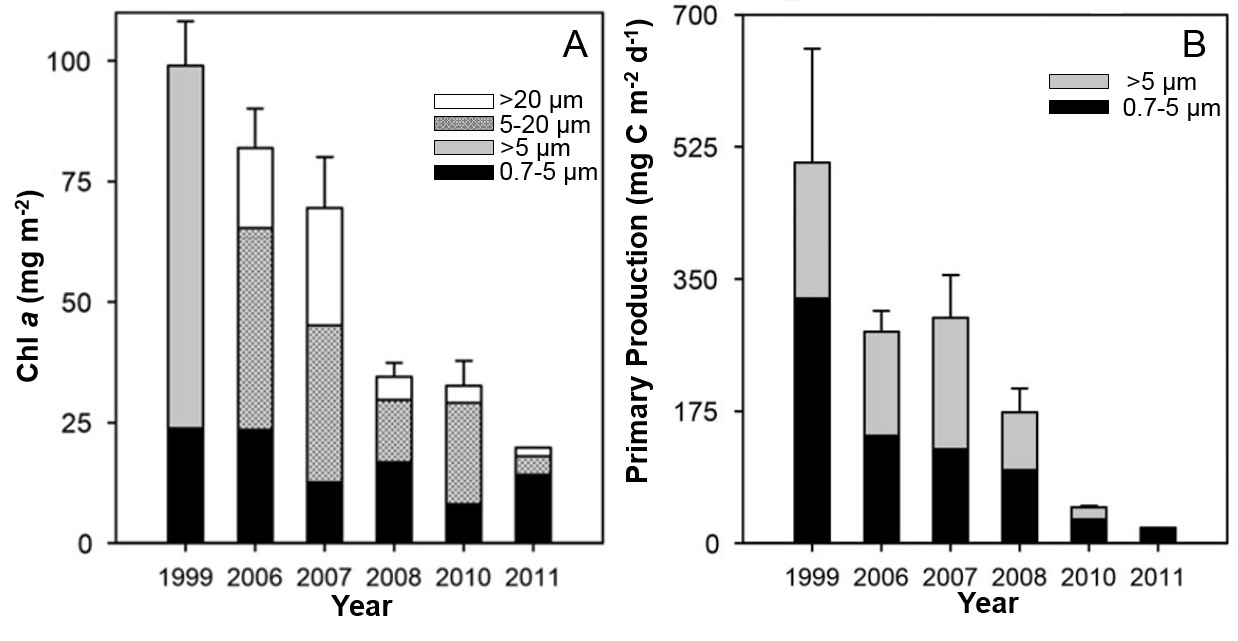
Figure 13. Time series of size-fractionated chlorophyll a (Chl a) biomass (a) and primary production (b) from 1999 to 2011, during fall in Baffin Bay. Data were integrated over the euphotic zone (down to 0.2% surface irradiance). In 1999 only two size fractions were measured. Values are mean ± 0.5 SE. In (b), the production by large cells is 3.2 mg C m-2 d-1 for fall 2011 (source: Blais et al. 2017).
Long description
Two bar graphs related to primary producers during fall in Baffin Bay. Plot A shows mean (± 0.5 standard error) size-fractionated chlorophyll a biomass on the y-axis (side), ranging from 0 to ca. 100 milligrams per meter squared for the years 1999, 2006, 2007, 2008, 2010, and 2011 (bottom, x-axis). For 1999 two phytoplankton size fractions are presented as different shaded parts of the bar, 0.7-5 micrometer and > 5 micrometer. For the other six years three size fractions are shown, 0.7-5, 5-20 and >20 micrometer. There is a decreasing trend in total chlorophyll a across the years. Trends in the size-classes are not explained in the text.
Plot B shows mean (± 0.5 standard error) size-fractionated primary production on the y-axis (side), ranging from zero to ca. 700 milligrams of carbon per meter squared per day, for the same years as in Plot A. The bars include dark and light shading to represent primary production attributed to two size fractions of phytoplankton (0.7-5 and >5 µm). There is a decreasing trend in total primary production across the years. Trends in the size-classes are not explained in the text.
Box: Range expansions
Range Expansions and New Species Occurrences
Karen Dunmall and Cory Matthews, Fisheries and Oceans Canada

Figure 14. Charlie Erigaktoak and Danny Gordon Jr. with a salmon they harvested in 2016 at Shingle Point, Yukon (photo credit: Michelle Gruben).
The Arctic is inherently dynamic, seemingly isolated, exceedingly vast, and some areas are experiencing rapid changes in biodiversity that have both local and global implications. Indeed, climate change is currently the most significant over-arching threat to current biodiversity in the Arctic (CAFF 2013), as the north is perceived both as a global conservation haven for species shifting distributions northward (Yoon et al. 2015), and a potential global conservation hazard for cold-adapted species in a warming environment (Reist et al. 2006a). The importance of assessing biodiversity is rooted in reconciling the emerging potential of the Arctic with protection of its environment, its species, and its Indigenous cultures.
Biologically, culturally and economically relevant indicator species provide information to assess ecosystem-level implications of on-going environmental changes in the Arctic. They also serve as a guide to the development of emerging opportunities. To be an effective indicator of a changing Arctic, however, species must be both sensitive to, and reflective of, environmental change within a defined period of time, detectable across a remote area and above ambient variability, scientifically based but not necessarily scientifically derived, and be relevant to the broader issues (DFO 2015).
Pacific salmon (Oncorhynchus spp.) are ideal harbingers of change because they have been identified as indicators of ocean status (Irvine and Riddell 2007), are responding to changing environmental conditions (Grebmeier et al. 2006; Dunmall et al. 2013; Nielsen et al. 2013), and can be tracked at northern distributional extremes using an established community-based monitoring program (Dunmall et al. 2013) (Figure 14 and Figure 15). The distributions of salmon species can reflect shifting environmental conditions both directly because fish, as ectotherms, maintain thermal preferences through behavioural choices (Reist et al. 2006b), and indirectly through the link between increased productivity and prey availability for potentially colonizing species (Dunmall et al. 2013). While salmon are not new to the Arctic (reviewed in Nielsen et al. 2013), current increases in abundance and distribution of salmon in the Arctic likely reflect broader-level changes. Salmon are a unique indicator of connectivities among oceans, countries, cultures, economies, and ecosystems.
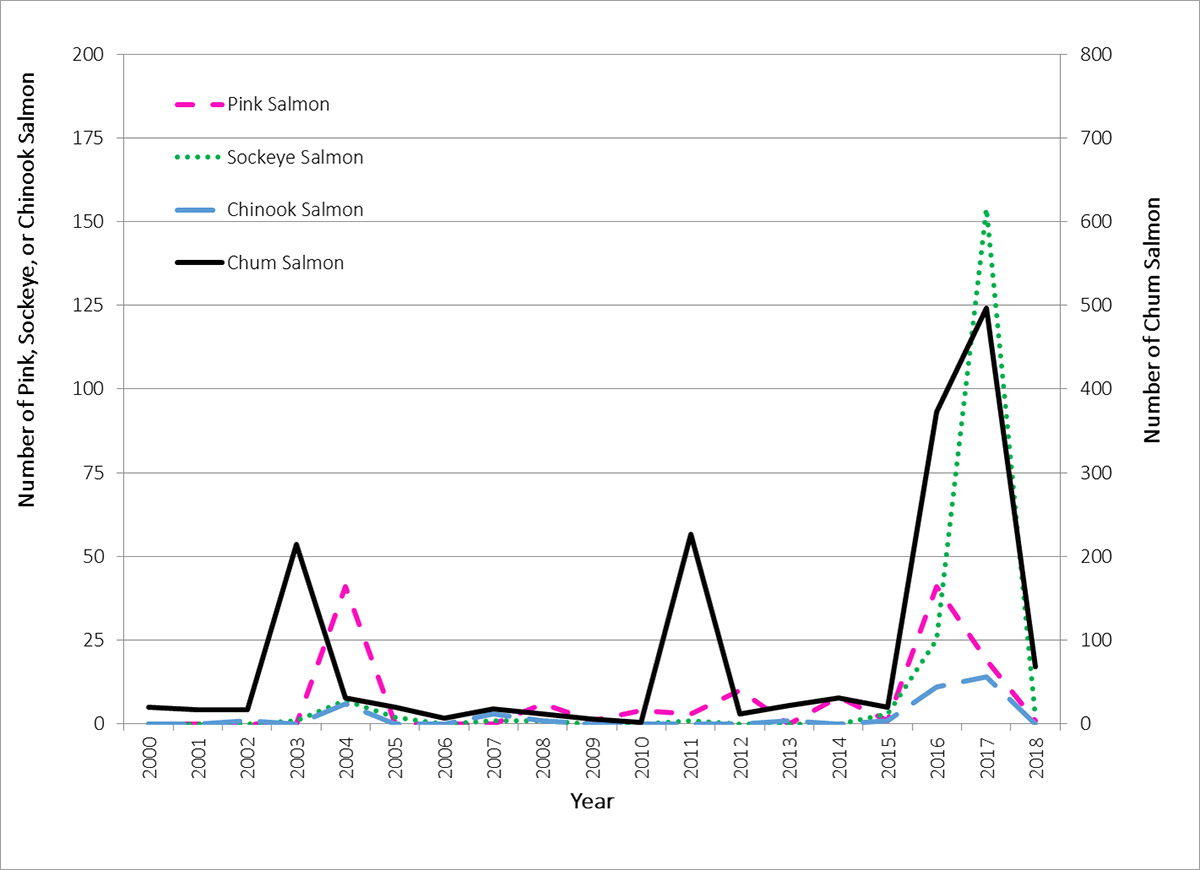
Figure 15. The number of each species of Pacific salmon received by the Arctic Salmon community-based monitoring program from harvesters across the Canadian Arctic from 2000–2017. Only one coho salmon was reported during this period (in 2011), thus it is not included (source: modified from Dunmall et al. 2018).
Long description
Line graph of the number of each species of Pacific salmon (side y-axis) received by the Arctic Salmon community-based monitoring program from harvesters across the Canadian Arctic from 2000 to 2017 (bottom, x-axis). The number of pink, sockeye or chinook salmon range from near zero to slightly more than 150 individuals. The number of chum salmon range from near zero to almost 500 individuals. Chum salmon numbers are around 25 for most years with increases to just over 200 individuals in 2003 and 2011 and a large increase in 2016 (ca. 395 individuals) and 2017 (500 individuals). Pink salmon numbers are less than 5 in most years, except for a peak of ca. 35 individuals on 2004 and a slighter higher peak in 2016. Chinook salmon numbers are also less than 5 in most years, increasing to ca. 10-15 individuals in 2016 and 2017. Sockeye Salmon show a very large increase to 150 individuals in 2017. All species show declines in 2018 relative to 2017. Only one coho salmon was reported during this period (in 2011), it is not included in the graph.
Killer whales (Orcinus orca) also represent connectivity between and changes within Canada’s oceans. In the eastern Canadian Arctic, killer whales (Figure 16) occur seasonally from about July to October, when the open water allows them to enter bays and inlets in search of marine mammal prey. Throughout their range from northern Baffin Island to southern Hudson Bay, killer whale sightings have increased over the past several decades (Higdon and Ferguson 2009; Higdon et al. 2013). Although killer whales are distributed worldwide and occur in relatively high densities at high latitudes, they are generally ice-avoidant in the Arctic (Matthews et al. 2011). The recent increases in sightings have been correlated with concurrent reductions in sea-ice extent and duration, with previously ice-covered areas that acted as barriers now opening up and allowing killer whale passage into areas where they have historically been rarely or never observed (Higdon and Ferguson 2009). Inuit in the eastern Canadian Arctic have reported that killer whale presence is increasing throughout the region (Higdon et al. 2013). Hudson Bay in particular has had an almost exponential increase in the number of killer whale sightings over the past several decades at the same time the region has experienced drastic sea-ice reductions (Higdon and Ferguson 2009). Although sightings data, separate from organized surveys, are subject to bias such as increased reporting awareness, it seems likely the greater numbers of sightings reflect a distribution shift, higher numbers, or a combination of both.
The consequences of an expanding Arctic killer whale presence with diminishing Arctic sea ice have been the focus of research at DFO over the past decade. Compilations of Inuit Ecological Knowledge and historical sighting reports that involve predation events indicate marine mammals are the primary, if not only, prey of Arctic killer whales (Higdon et al. 2013; Westdal et al. 2016a). Modelling exercises suggest mortality from killer whale predation could potentially be high enough to impact other Arctic marine mammal populations (Ferguson et al. 2012). Other recent studies led by DFO have shown the negative effects of killer whale predation extend beyond direct mortality of prey. Killer whales, for example, have a strong impact on the behaviour and distribution of narwhals. In the absence of killer whales, narwhals preferred to be in open, deep waters, while in their presence, narwhals preferred shallow, nearshore areas (Breed et al. 2017). Although this behaviour has been long known to Inuit, this study was unique in that it showed the narwhal response was sustained for as long as they shared the relatively large inlet with killer whales, and persisted beyond discrete predation events. Bowhead whale (Balaena mysticetus) behaviour and selection of sea-ice habitat is also greatly impacted by killer whale presence. Bowhead whales in the Gulf of Boothia also preferred open-water habitat in the absence of killer whales, but rapidly retreated into ice cover and close to shorelines when killer whales were present (C. Matthews, unpublished data). Again, the behaviour was exhibited gulf-wide by all tagged bowheads for the duration of time killer whales were present, representing a response that had never been documented for marine vertebrates on such a large scale. The pronounced killer whale-mediated shifts in the behaviour and habitat use of Arctic marine mammal prey could translate into costly non-consumptive effects through lost foraging opportunities or increased stress, potentially having a negative impact on prey populations that stands to be exacerbated by climate change (Breed et al. 2017; C. Matthews, unpublished data).
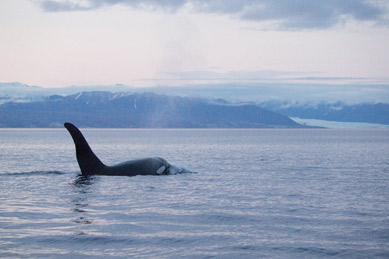
Figure 16. Killer whale in Eclipse Sound, Nunavut in summer 2018 (photo credit: Maha Ghazal).
Long description
Photograph of a killer whale swimming in Eclipse Sound, Nunavut in summer 2018. Snow covered mountains can be seen in the background. The large dorsal fin is above the water and blow from the whale is visible in the sky.
Anticipated killer whale range expansions in the Arctic, however, may not be so clear-cut. At the same time killer whale sightings have increased in the eastern Canadian Arctic, so too have the number of ice entrapments involving killer whales. Hidgon and Ferguson (2014) and Westdal et al. (2016b) report on several fatal killer whale ice entrapments in the eastern Canadian Arctic since the early 1950s, which is more than the reported number of similar events over the previous century. During the most recent of these, in 2016, killer whales were observed overwintering in southeast Hudson Bay, but were found dead the following spring. Matthews et al. (2019) speculated that the whales entered Hudson Bay in pursuit of beluga the previous summer, but failed to exit prior to ice formation and died of starvation after being unable to meet energetic requirements throughout the winter. The four confirmed deaths from the most recent event combined with the deaths from the previous ice entrapments represent a significant portion of the estimated population of killer whales that occur in the eastern Canadian Arctic (Young et al. 2011). Killer whale ice entrapments are almost always fatal and can wipe out entire family groups, with long-lasting demographic impacts (Higdon et al. 2013). Ice entrapments could therefore slow Arctic killer whale range expansions, particularly in areas where killer whales that are unfamiliar with sea-ice patterns fail to exit prior to ice formation in winter (Matthews et al. 2019).
Box: Arctic hotspots
Marine Mammal Hotspots: Focal Points of Energy Flow Within the Canadian Arctic
Dave Yurkowski, Fisheries and Oceans Canada
Quantifying biogeographical attributes, such as Arctic marine predator movements and their distributions, has important implications for understanding how the Arctic ecosystem is structured and how it functions (Moore and Huntington 2008). Highly mobile marine predators (e.g., marine mammals, seabirds and large fishes) integrate resources across numerous spatial and temporal scales, therefore can act as sentinels for areas of high productivity and how this changes seasonally in highly dynamic environments such as the Arctic (Boyce et al. 2015). Observing animals within the dynamic nature of Arctic marine environment is difficult, but the application of animal telemetry devices has revolutionized our understanding of the movement ecology of marine species (Hussey et al. 2015). Traditionally, telemetry studies on Arctic marine predators have focused on single or a few species, but given the amount of telemetry data currently available for Arctic marine predators one can now quantify species diversity hotspots, and infer specific areas of higher biological importance (i.e., hotspots) during summer-autumn and winter-spring.
A compilation of existing animal tracking data was collected between 1989 and 2016 for 1,283 individuals of 21 iconic Arctic marine species across cetacean, pinniped, seabird, polar bear (Ursus maritimus) and fish species groups. Some iconic species include: belugas (Delphinapterus leucas), narwhals (Monodon monoceros), ringed seals (Pusa hispida), Atlantic walrus (Odobenus rosmarus rosmarus), common eiders (Somateria mollissima), northern fulmars (Fulmarus glacialis), thick-billed murres (Uria lomvia), and Greenland sharks (Somniosus microcephalus). Hotspots were generally along the continental shelf and slope throughout summer-autumn and were generally offshore in known areas of moving pack ice during winter-spring, therefore generally correspond to seasonal productivity patterns (Figures 17 and 18). These movements exhibit seasonal connectivity and connectivity between offshore and nearshore energy channels during winter-spring and summer-autumn, respectively. Specifically, in the west, nutrient-rich waters from the Pacific Ocean and Bering Sea flow northward through the Bering Strait and southern Chukchi Sea leading to enhanced pelagic and benthic faunal biomass in the area and along the continental shelf and shelf break to the Mackenzie Delta (Grebmeier et al. 2006). During winter-spring, hotspots occurred in productive areas westward of the Cape Bathurst Polynya. In the East, Baffin Island consists of many productive fjords due to increased organic carbon content in the water column (Syvitski et al. 1990). During winter, species diversity hotspots were concentrated in dense mobile pack ice areas of Baffin Bay and Davis Strait. In the South, species diversity hotspots coincided with productivity patterns of the Hudson Bay complex (Harvey et al. 2006). And overwintering hotspots were within the moving pack-ice and open-water areas of Hudson Strait. Overall, hotspots in the winter highlight the ecological importance of polynyas and pack ice areas to Arctic ecosystem structure and function (Stirling 1997). As such, identifying areas where predator densities are highest provides critical information for understanding the dynamics of energy flow throughout the Arctic and thereby demonstrates the importance of connectivity for conservation efforts.
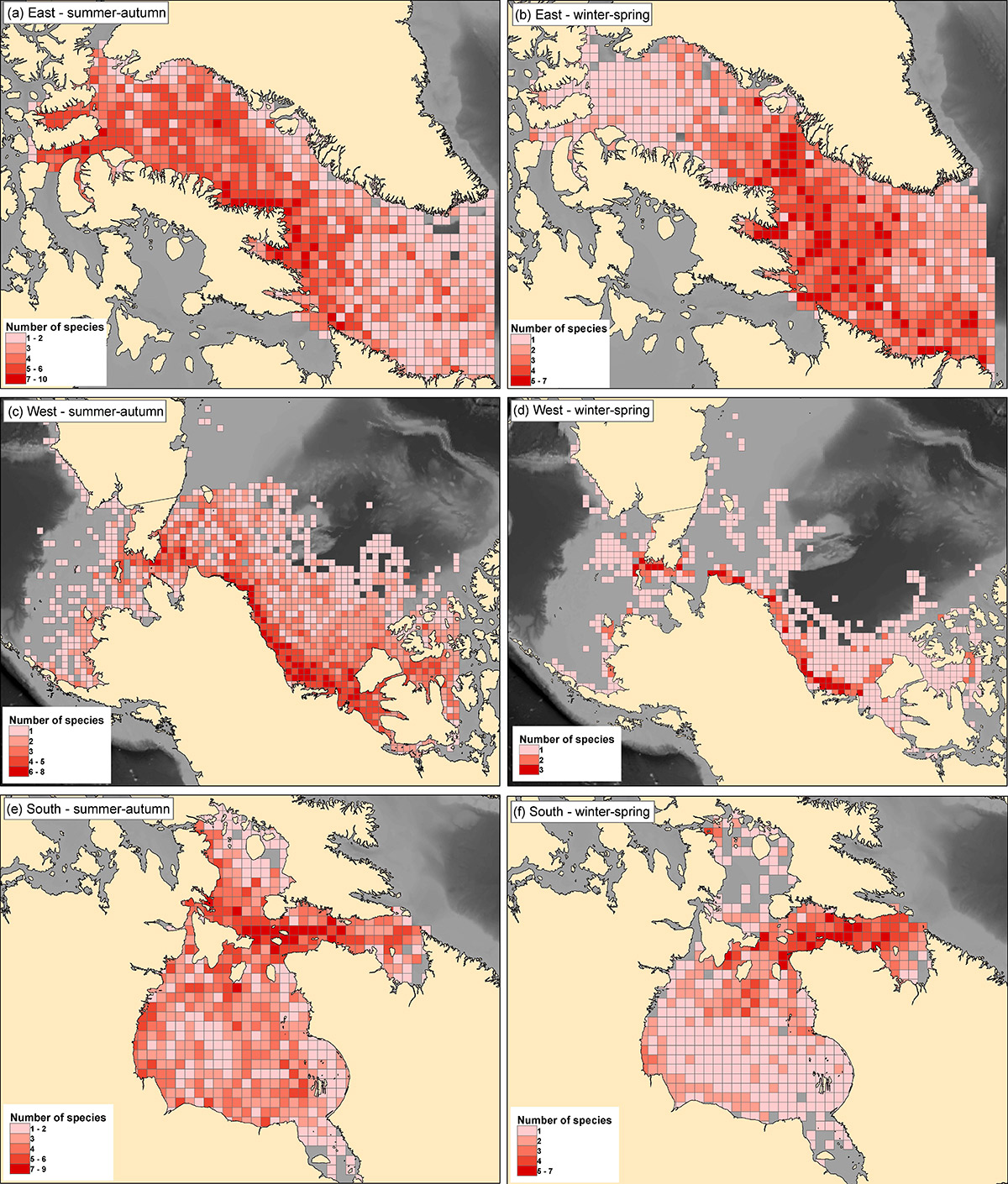
Figure 17. Spatial distributions of density of unique number of species across 21 species per 50 km x 50 km grid cell within each of the three geographic areas during summer-autumn (a, c, e) and winter-spring (b, d, f). The legend for winter-spring is different from summer-autumn, as is the legend between each geographic area (source: Yurkowski et al. 2019).
Long description
The figure contains plots from three geographical areas in the North American Arctic during summer-autumn (plots a, c and, e) and winter-spring (plots b, d and, f). The plots show the spatial distribution, represented by a light to dark red color scale, for the density of unique number of species across 21 species per 50 kilometre x 50 kilometre grid cell. The legend indicating the number of species per color shade, for winter-spring is different from summer-autumn, as is the legend between each geographic area. Plots A and B show the marine area between Baffin Island and Greenland, extending down past Labrador and including Lancaster Sound in the north. In summer-autumn the highest density of tracked individuals is located in the northern portion of the area, extending along the east coast of Baffin Island. In the winter-spring, highest densities are found around the southern end of Baffin Island, extending across to Greenland. Plots C and D show the marine area on the Alaskan and Russian shelf, the Bering Strait, Chukchi and Beaufort Seas and includes waters around Banks and Victoria Islands in the Canadian Arctic. In summer-autumn the area is widely used and highest densities are found along the Chukchi and Beaufort Shelf area as well as Prince of Wales Strait. In winter-spring, a smaller portion of the area is used and highest densities are located very near the North American continental mainland. Plots E and F cover Hudson and James Bay as well as Foxe Basin and Hudson Strait. The area is widely used with high densities extending into Hudson Bay from Hudson Strait in both seasons. The high densities are more constrained to Hudson Strait during winter-spring than in summer-autumn.
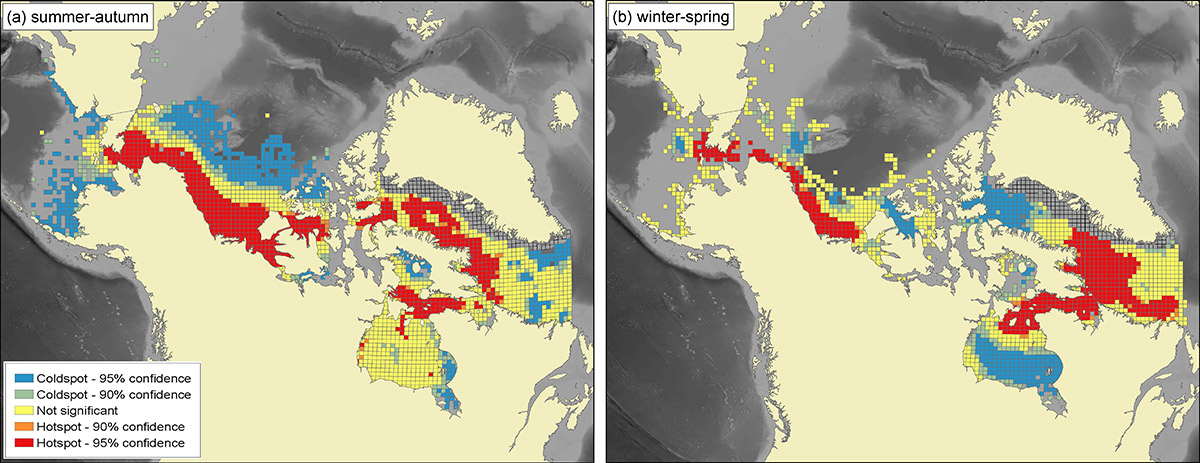
Figure 18. Species diversity hotspots (red) and coldspots (dark blue) by summer-autumn (a) and winter-spring (b) of all tracked species per 50 km x 50 km grid cell across the study area. Grid cells along the West Greenland continental shelf have been masked due to a lower number of tagging locations in these areas than in Canadian waters resulting in less confidence in identifying hotspots and coldspots along the West Greenland coast (source: Yurkowski et al. 2019).
Long description
Two maps of the North American Arctic showing species diversity hotspots (red) and coldspots (dark blue) by summer-autumn (a) and winter-spring (b) of all tracked species per 50 km x 50 km grid cell across the study area. Grid cells along the West Greenland continental shelf are not included (i.e., masked) due to a lower number of tagging locations in these areas than in Canadian waters resulting in less confidence in identifying hotspots and coldspots along the West Greenland coast. Not significant areas are indicted by yellow shading. In summer-autumn (Plot A) there is a hotspot in the western Arctic extending from M’Clure Strait westward along the Mackenzie and Chukchi shelf and slope, ending in the Bering Strait. Other hotspots include Hudson Strait, Lancaster Sound and areas along the eastern side of Baffin Island. Cold spots include deep areas in the Canadian Basin as well as Alaskan waters and James Bay. In winter-spring (Plot B), the western Arctic hotspot remains but it is much reduced, hugging the shelf area next to the Canadian and Alaskan mainland. The hotspot area in the eastern arctic is much more concentrated, centering around the southern part of Baffin Bay, and branching through Hudson Strait and into the northern part of Hudson Bay. The hotspot also branches southward just offshore of the Labrador coast. Northern Baffin Bay, M’Clure Strait and southern Hudson Bay are cold spots. The hot and coldspots are presented with 95% confidence.
Box: Community monitoring
Linking Traditional Ecological Knowledge, Western Science, and Environmental and Fisheries Management in the Western Canadian Arctic
Burton Ayles, Fisheries Joint Management Committee
The comprehensive land claims in the Canadian Arctic recognize the rights of the Inuit to the renewable resources of the region and the right to participate in the management of those resources. They also recognize the traditional cultures of the Inuit, and that Inuit knowledge and practices need to be reflected in management of the wildlife. Governments have responded to these agreements, and to numerous court cases by modifying laws, policies and practices to ensure that Indigenous knowledge, as well as Western-based science helps to support resource management decision making. But the way forward to translate the numerous words into the realities of everyday actions in the management and protection of fisheries and wildlife and their associated habitats is far from clear (e.g., Laidlaw 2015).
In recent years there have been continued efforts to document and catalogue ecosystem Traditional Ecological KnowledgeFootnote 1 (TEK) (e.g., ISR Traditional and Local Knowledge Catalogue, Hudson Bay SIKU, Nunavut Coastal Inventory, Byers et al. 2019). Here we describe an environmental and fisheries management model (Dorcey and Hall 1981) that is expanded to include TEK. It is presented from the perspective of the Inuvialuit Settlement Region (ISR) (Canada 1984) but could be applied elsewhere.
The model (Figure 19) is a visualization of the link between knowledge generation (TEK and Western Science) and environmental and fisheries management decision-making within the co-management framework established by the land claims. The intention is to provide an approach to incorporating TEK as a useful tool of fisheries and environmental management by recognizing the importance of the full spectrum of Inuit understanding of the environment.
Environmental and fisheries management decisions can be viewed as a spectrum or continuum from single purpose, to complex multi-purpose, or integrated decisions. The bottom horizontal arrow in Figure 19 shows this continuum and examples of the decision processes from the ISR that are regularly invoked in the Canadian Arctic. Community Conservation Plans (CCPs) (EISC 2018), developed by the Hunters and Trappers Committees (HTCs), are key processes for environmental decision making. CCPs reflect community values and goals and categorize land and resource uses for the area. Scientific information is included to complement the TEK but the CCP origins are within the communities and then are linked directly to the planning of proposed industrial development. The diverse management processes on the decision continuum are supported by research activities that span the spectrum from descriptive (e.g., what species and where) to functional knowledge (e.g., system relationships such as how seal pup survival varies with ice formation or how char populations will respond to fishing).
In the model, the black vertical arrows demonstrate the link between the scientific knowledge spectrum and the management decision spectrum. The relative position of the five research activities and the management decisions is important. Descriptive knowledge contributes primarily to the single objective, single purpose end of the management decision-making spectrum while new functional knowledge is essential for integrated decisions but also contributes to the other end of the spectrum. Dorcey and Hall (1981) argue that if management is to be improved, decision making should move to the right with greater emphasis on support for enhancing functional knowledge and thus specific hypothesis testing.
TEK also can also be viewed as a spectrum of complexity from simple individual observations, to community governance and knowledge, to world views and values. Along the top horizontal arrow some activities supported by co-management with respect to TEK are presented as a continuum of complexity. The vertical blue arrows show linkages and parallels between the TEK and science spectrums and then indirectly to the management spectrum. There are also direct linkages (vertical blue arrows) from the TEK spectrum to the management spectrum. Five examples are provided to illustrate TEK flow through the management model. The blue arrow on the far right identifies that Inuit stories, beliefs and cultural practices can directly affect management decisions. In the western Arctic influential elders including Alex Aviugana and Billy Day helped negotiate the Inuvialuit Final Agreement (IFA) (Canada 1984) and served with effectiveness and distinction on many Inuvialuit and co-management organizations bringing their traditional knowledge to the negotiations framed primarily by a western bureaucratic public administration (Beck 1994; Bell 2009). More recently Inuit are speaking via Facebook, Twitter and videos documentaries directly to a broader public to explain their lives and culture. In “Angry Inuk” Inuit director Alethea Arnaquq-Baril (Arnaquq-Baril 2016) challenges the EEC (European Economic Community) ban on commercial seal harvests. In so doing she has helped to raise awareness of Europeans and other North Americans to Inuit desire for a sustainable economy and food source based on traditional resources of fish and marine mammals rather than welfare and imported southern produce. While the campaign was ultimately unsuccessful, it is approaches like this that can lever the world view of some 132,000 Inuit to help influence public attitudes of over one billion people in Europe and North America to enhance environmental sustainability and protection for the entire Arctic.
The model is open to the criticism that it is a typical Western bureaucratic approach to the integration of TEK into management and does not adequately reflect the real differences between aboriginal cultures and the main stream view of the world. As Stevenson (2004) has argued, this approach may just be seen as further appropriation of TEK into Western scientific thought and practice. This may be so but the reality is that the co-management system in the Arctic is primarily a Western state system that requires the full spectrum of both TEK as well as Western science. The proposed model highlights potential relationships between the spectrums of knowledge and how, together, they can contribute to the range of practices required for environmental and fisheries management in the Arctic. Functioning under such model should facilitate communication between participants (e.g., harvesters, scientist, politicians) and help focus TEK and western science efforts on specific activities required to improve decision making.
The model presented here, implemented within a co-management framework, offers promise to enhance the interface between TEK and Western science and environmental and fisheries management. Paraphrasing White (2006), co-management boards in the Arctic are substantively important in terms of gauging the influence of TEK and represent the best opportunity for imbuing public, non-Aboriginal governmental institutions with TEK. In other words if co-management boards cannot successfully integrate TEK into their processes and decisions it is unlikely that another public institution would fare any better.
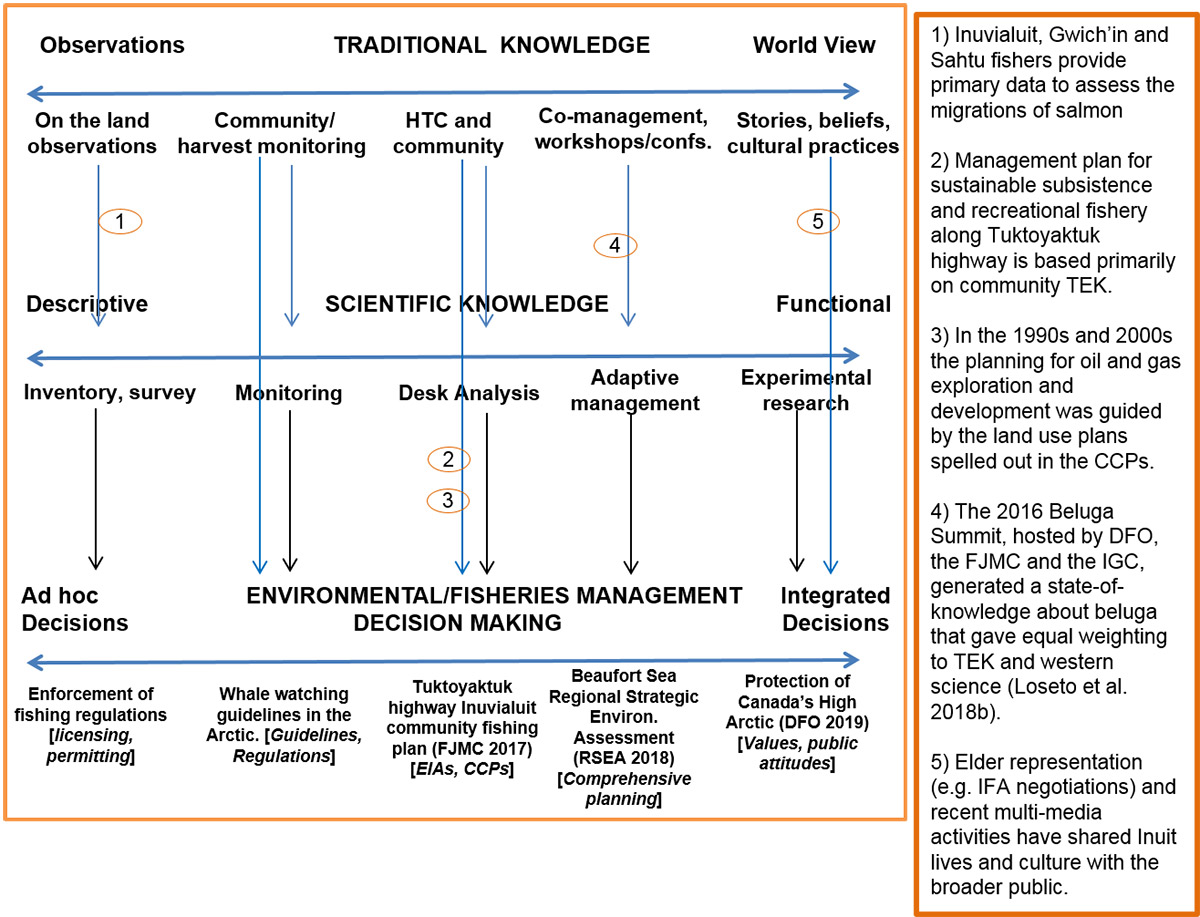
Figure 19. A visual model of the relationship between TEK, Western Scientific Knowledge and Environmental/Fisheries Management Decision-Making. EIA: Ecosystem Impact Assessment; FJMC: Fisheries Joint Management Committee; IGC: Inuvialuit Game Council.
Long description
A visual model of the relationship between Traditional Ecological Knowledge (TEK), Western Scientific Knowledge and Environmental/Fisheries Management Decision-Making. The model includes three horizontal blue arrows that represents a spectrum of complexity for 1) Traditional Knowledge which spans from observations (right) to World View (left). Examples along this spectrum are “On the land observations”, “Community/harvest monitoring”, “Hunter and Trapper Committee (HTC) and community”, “Co-management workshops/conferences”, and “Stories, beliefs, cultural practices”. The second horizontal blue arrow describes the spectrum of Scientific Knowledge, ranging from Descriptive (left) to Functional (right). Examples along this spectrum are “Inventory, survey”, “Monitoring”, “Desk Analysis”, “Adaptive management” and, “Experimental research”. The third horizontal blue arrow describes the spectrum of Environmental/Fisheries Management Decision Making ranging from Ad hoc Decisions (left) to Integrated Decisions (right). Examples along this spectrum are “Enforcement of fishing regulations [licensing, permitting]”, “Whale watching guidelines in the Arctic [Guidelines, Regulations]”, Tuktoyaktuk highway Inuvialuit community fishing plan [Ecosystem Impact Assessments, Community Conservation Plans]”, “Beaufort Sea Regional Environmental Assessment [Comprehensive planning]”, and, “Protection of Canada’s High Arctic [Values, public attitudes]”.
Black vertical arrows demonstrate the link between the scientific knowledge spectrum and the management decision spectrum. Each example along the Scientific Knowledge spectrum is connected to corresponding position along the Environmental/Fisheries Management Decision Making spectrum.
Blue vertical arrows demonstrate how TEK links to the science spectrum and then indirectly to the Management spectrum. There are also direct linkages between the TEK and the Management spectrum. Five example are provided.
- 1) Link between “On the land observations” and science “Inventory, survey”: Inuvialuit, Gwich’in and Sahtu fishers provide primary data to assess the migrations of salmon.
- 2 and 3) Link between “HTC and community” and decision making using EIAs and CCPs:
- Management plan for sustainable subsistence and recreational fishery along the Tuktoyaktuk highway is based primarily on community TEK.
- In the 1990s and 2000s the planning for oil and gas exploration and development was guided by the land use plans spelled out in the CCPs.
- 4) Link between “Co-management workshops/conferences” and science “Adaptive management”: The 2016 Beluga Summit, hosted by DFO, the Fisheries Joint Management Committee and the Inuvialuit Game Council, generated a state-of-knowledge about beluga that gave equal weighting to TEK and western science.
- 5) Link between “Stories, beliefs, cultural practices” and management “Integrated Decision”: Elder representation (e.g. Inuvialuit Final Agreement negotiations) and recent multi-media activities have shared Inuit lives and culture with the broader public.
Citizen Science, Arctic Style
Karen Dunmall and Kim Howland, Fisheries and Oceans Canada
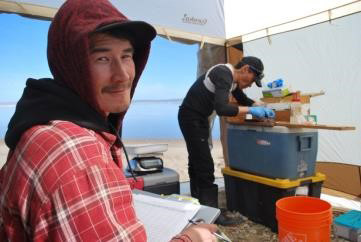
Figure 20. Brandon Green and Steve Illasiak processing fish for samples and recording biological data in Anguniaqvia niqiqyuam marine protected area, Northwest Territories, 2018 (photo credit: Darcy McNicholl).
The rapidly changing Arctic presents challenges to both scientists assessing impacts and opportunities, and to Indigenous Peoples who are intimately connected to the environment and its resources. Arctic biodiversity is irrevocably linked to the culture of Indigenous Peoples in the north and their perceptions of change are rooted in their dependence on the environment and social values to protect subsistence opportunities (CAFF 2013). Indeed, the dearth of scientific information about species and their habitats in the Arctic (Reist et al. 2006a) is contrasted with the breadth of Inuit knowledge about the environment. Therefore, innovatively creating the opportunity to assess change by those directly observing immediate effects provides a powerful approach to facilitate human influence on the pace of science as well as our role in the pace of change. Citizen science in the Canadian Arctic can be an important community-based monitoring tool that bridges Indigenous knowledge and scientific research, and ultimately results in knowledge co-production (Dunmall and Reist 2018).
The commonality of using specific and easily documented indicators to assess environmental change in both Indigenous and scientific knowledge systems provides the foundation for citizen science in the Canadian Arctic (Dunmall and Reist 2018). The contemporary knowledge obtained by monitoring the environment through indicators can be translated to developing baseline data and quantitatively monitoring ecological changes at both fine- and broad-spatial scales. Although the broad suite of indicators usually gathered by Indigenous knowledge is often different than the specific indicators used in scientific research, both of these monitoring methods are linked and thus can highlight similar ecosystem-level changes (Riedlinger and Berkes 2001; Berkes et al. 2007; Tremblay et al. 2008). Communication and outreach is vital to the success of citizen science in the Arctic, and is facilitated in part through the use of social media (e.g., Facebook) to distribute information and interact with participants and observers (Dunmall and Reist 2018).
The prevalence of community-based monitoring is increasing in the Canadian Arctic. The Arctic Salmon program (www.facebook.com/arcticsalmon) is using a community-based monitoring approach to track increasing abundances and broadening distributions of Pacific salmon (Oncorhynchus spp.) and distributional shifts of fishes in the Canadian Arctic (Dunmall et al. 2013, 2017, 2018). Harvest-based monitoring of beluga whales (Delphinapterus leucas) provides important data about beluga health and condition based on the provision of samples and measurements of specific indicators (Loseto et al. 2018c). Community-based monitoring of the physical environment, including the collection of Inuit knowledge is also occurring in Hudson Bay (e.g., Arctic Eider Society https://arcticeider.com/siku). Community-based monitoring of the coastal ecosystem, including data collections on environmental parameters, primary production, benthic habitats, and fish biodiversity has been on-going in Darnley Bay, Northwest Territories, since 2012 (McNicholl et al. 2017) and was recently developed into a community-based monitoring framework tested for its transferability in the coastal environments near Kugluktuk, Nunavut in 2017 and Sachs Harbour, Northwest Territories in 2018 (McNicholl and Dunmall 2018a). In communities with relatively high shipping activity, and thus at higher risk for invasive species, techniques for community-based port surveys and environmental DNA (eDNA) sampling has been introduced (2015-18: Churchill, MB, Salluit (Nunavik), Iqaluit and Pond Inlet (Nunavut)) (Polar Knowledge Canada 2017). These efforts have been combined with youth focused educational workshops to raise awareness and provide identification guides/hands on training in how to report new sightings and distinguish high risk invasive species from similar native species.
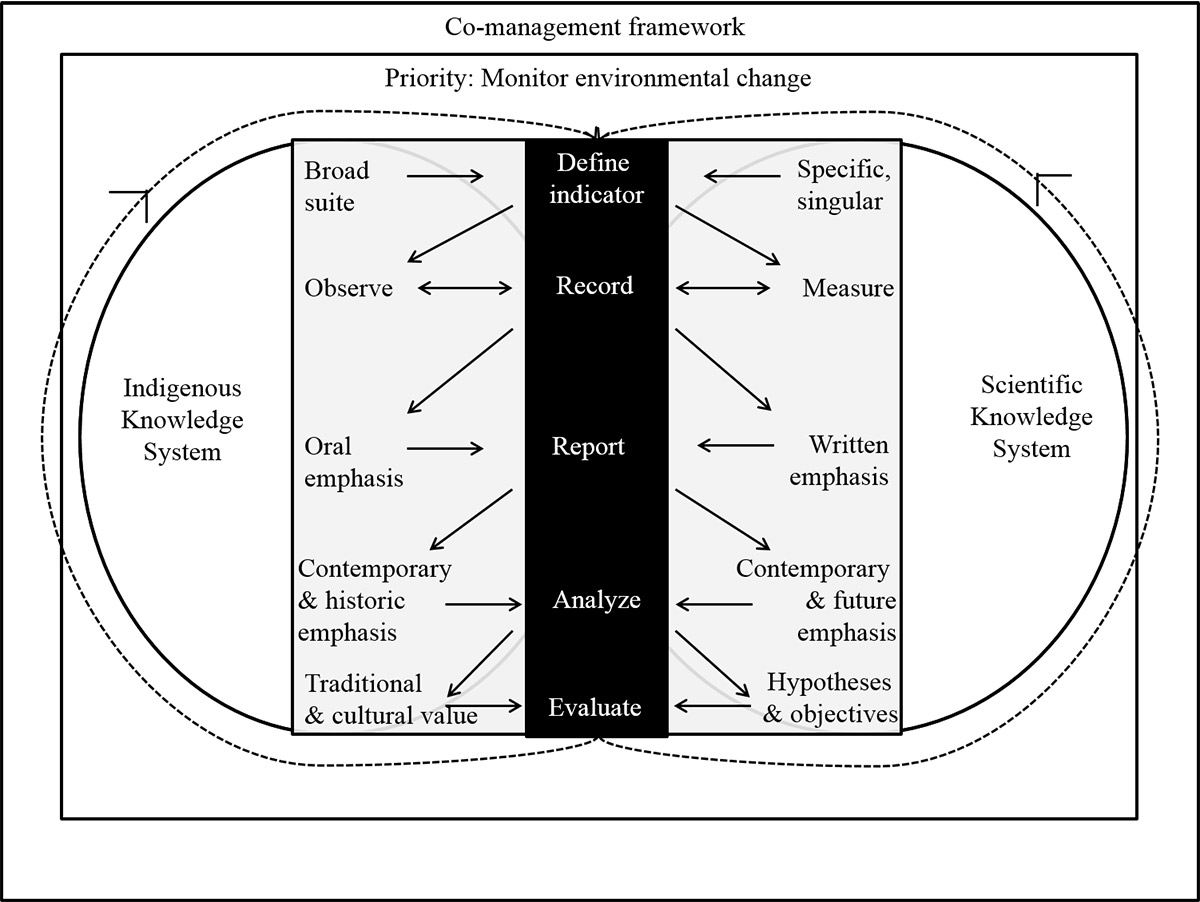
Figure 21. A model for citizen science to monitor a changing Canadian Arctic. Under a common priority of monitoring environmental change, citizen science (grey square) can bridge Indigenous and scientific knowledge systems (white circles). The arrows depict the contributory flow of information from the general citizen science framework (black box) out to each knowledge system and back to the framework at each step in the process, which equalizes the value of information derived from indigenous knowing and scientific knowledge and contributes to knowledge co-production. Each step in the framework is described from the perspective of each knowledge system. A key element is the interactive reporting process and ongoing communications among participants (source: Dunmall and Reist 2018).
Long description
A descriptive (work and arrows) model of citizen science to monitor a changing Canadian Arctic. Under a common priority of monitoring environmental change, citizen science (grey square) can bridge Indigenous and scientific knowledge systems (indicated by white circles). Citizen science under an Indigenous knowledge system can be described as/include: broad suite, observe, oral emphasis, contemporary & historic emphasis, traditional & cultural value. In comparison citizen science under a Scientific knowledge system can be described as/include: Specific/singular, measure, written emphasis, contemporary & future emphasis, hypothesis & objectives.
Arrows depict the contributory flow of information from the general citizen science framework (black box with steps: define indicator, record, report, analyze, evaluate) out to each knowledge system and back to the framework at each step in the process, which equalizes the value of information derived from indigenous knowing and scientific knowledge and contributes to knowledge co-production. A key element is the interactive reporting process and ongoing communications among participants.Community-led monitoring is now also emerging in the Canadian Arctic. This represents a subtle but very important shift to leadership roles by Indigenous Peoples in all aspects of field-based monitoring efforts within a collaborative framework rooted in science. Transitioning to this community-led effort is a process that requires time and effective communication, motivation, and mutual agreement on indicators and goals, as well as capacity building through the provision of protocols, gear, and training. Community-led monitoring efforts in Anguniaqvia niqiqyuam Marine Protected Area, near Paulatuk, Northwest Territories, successfully collected data in 2018 on indicators spanning multiple trophic levels, as well as environmental data regarding the coastal ecosystem (McNicholl and Dunmall 2018b) (Figure 20). Leadership in beluga whale monitoring has been consistent among Inuvialuit and the recent collection of TEK (Ostertag et al. 2018; Waugh et al. 2018) complements these efforts by providing ecosystem-level context to specific indicators (Loseto et al. 2018c).
Citizen science is broadly appealing as a tool to assess environmental change in the Arctic. When carefully applied in a model that emphasizes continuous effective communication and ensures the collaborative production of knowledge (Figure 21), citizen science can provide critical information necessary to predict impacts and opportunities associated with biodiversity shifts and rapidly changing ecosystems in the Arctic. Moreover, if eventually applied consistently using a common framework, citizen science delivered by Indigenous Peoples provides wide geographic coverage and monitoring over the longer term, which will lead to better understanding of the nature, rates and consequences of Arctic change. This, in turn, will result in more effective resource co-management and environmental stewardship.
Box: Knowledge integration
Beluga Monitoring, Management, and Human Relations
Lisa Loseto, Elizabeth Worden, Fisheries and Oceans Canada
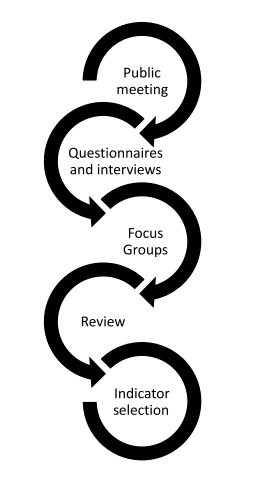
Figure 22. The process followed to select beluga health indicators with Inuvik, Tuktoyaktuk and Paulatuk.
Long description
Image depicts the process followed to select beluga health indicators with the communities of Inuvik, Tuktoyaktuk and Paulatuk. Each step (curved arrow) feeds into the next. The steps are public meting, questionnaires and interviews, focus groups, review, indicator selection.
Each summer thousands of Eastern Beaufort Sea (EBS) beluga, qilalukkaq (Delphinapterus leucas) whales aggregate in the Mackenzie Estuary of the Canadian Beaufort Sea. This population is recognized as one of Canada’s largest, last estimated at approximately 40,000 individuals (Harwood et al. 1996; Hill and Demaster 1999). The subsistence harvest of these beluga, by Inuvialuit, the Inuit of Canada’s Western Arctic, has, and continues to be of important economic, dietary, and cultural importance. EBS belugas are co-managed by the Fisheries and Oceans Canada (DFO) and the Fisheries Joint Management Committee (FJMC) under the guidance of the Inuvialuit Game Council (IGC) as per the Inuvialuit Final Agreement land claim (Canada 1984). The IFA, the Beaufort Sea Beluga Management Plan and earlier conservation management efforts supported the collection of beluga harvest data through partnerships among co-management bodies and in cooperation with the local Hunters and Trappers Committees (HTCs).
As a result, more than 40 years of data have been collected about the EBS beluga via the harvest monitoring program, a community-based monitoring (CBM) program (Harwood et al. 2002). The program has evolved to provide a centre-point to bring together scientists, community members and co-management boards to work as a team to address community concerns and identify research directions. In 2010, the beluga management zones identified as 1a became the first Arctic Marine Protected Area, the Tarium Niruitait MPA (Loseto et al. 2010). Part of the MPA monitoring and management framework involves the use of indicators that are used to measure and communicate the health of MPA. Over the last 10 years the existing beluga CBM grew in depth and breadth to include indicators on beluga condition, diet, health that included contaminant and disease monitoring and other physiological indicators that can also be used to extrapolate to ecosystem health (Loseto et al. 2018b). While the monitoring expanded to include multiple health and ecological indicators it was noted that the CBM program was lacking a mechanism to include the extensive Inuit knowledge, referenced as Local and Traditional Ecological Knowledge (LEK, TEK) held by Inuvialuit in the ISR.
Inuvialuit and their ancestors have sustainably managed the population for hundreds of years (McGhee 1988; FJMC 2013). Subsequently, the Inuvialuit have extensive knowledge about the behaviour and health characteristics of beluga whales in the ISR. Through long-term working relationships established through collaboration on various beluga-related projects, a project was initiated to address gaps in the integration of LEK and TEK in the beluga CBM program (S. Ostertag, unpublished data). Through an extensive consultation process (2012-2017), indicators of beluga condition and disease were identified by beluga knowledge-holders in the three most active beluga harvesting communities in the ISR; Inuvik, Tuktoyaktuk and Paulatuk (S. Ostertag, unpublished data). This process focused on Inuvialuit knowledge-sharing, frequent communication, and collaborative feedback through various stages of research (S. Ostertag, unpublished data) (Figure 22). Examples of indicators identified by the three communities include: the colour and texture of the fat/uqsuq, the shape of the body (broad or round back, fat rolls described as ‘love handles’) and signs of infection. These indicators have now been incorporated into the FJMC Fish and Marine Mammal Community Monitoring Program to ensure that Inuit observations about beluga health and condition are recorded for harvested beluga whales (Figure 23).
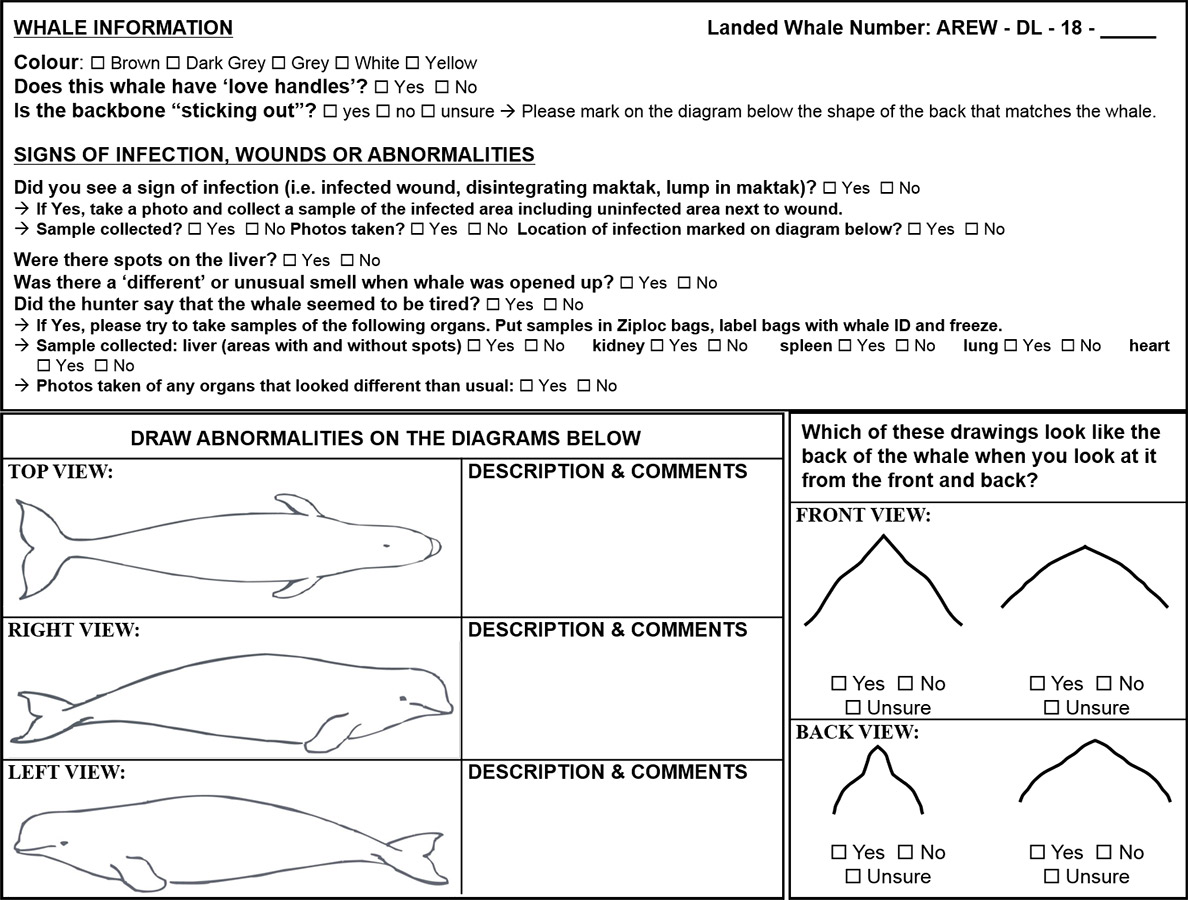
Figure 23. FJMC/DFO beluga monitoring sheet (1 of 2 sheets) that includes TEK based indicators.
Long description
A photo of the FJMC/DFO beluga monitoring sheet (1 of 2 sheets) that includes TEK based indicators. Information collected includes color (choices given), the presence of love handles (yes/no), and shape of backbone. Signs of infection, wounds or abnormalities are recorded. The sheet also includes a section where abnormalities can be drawn on whale diagrams (top, right or left view of the whale). There is also a section to indicate which drawing looks like the back of the whale when viewed from the front and back.
The success of the community-identified beluga health indicators is largely due to the repeated annual observations of active harvesters on the land and their interactions with beluga whales. Inuvialuit knowledge of the beluga whale hunt is dynamic with deep roots containing both traditional components, passed down through oral history, as well as current and ever-changing local observations. However, despite this intergenerational beluga whale harvest, recent changes – both environmental and social – have impacted human-beluga relations in the region. For example, Aklavik a community with a long history of active beluga harvesting, has experienced a sharp decline in the annual whale harvest (Worden 2018) (Figure 24).
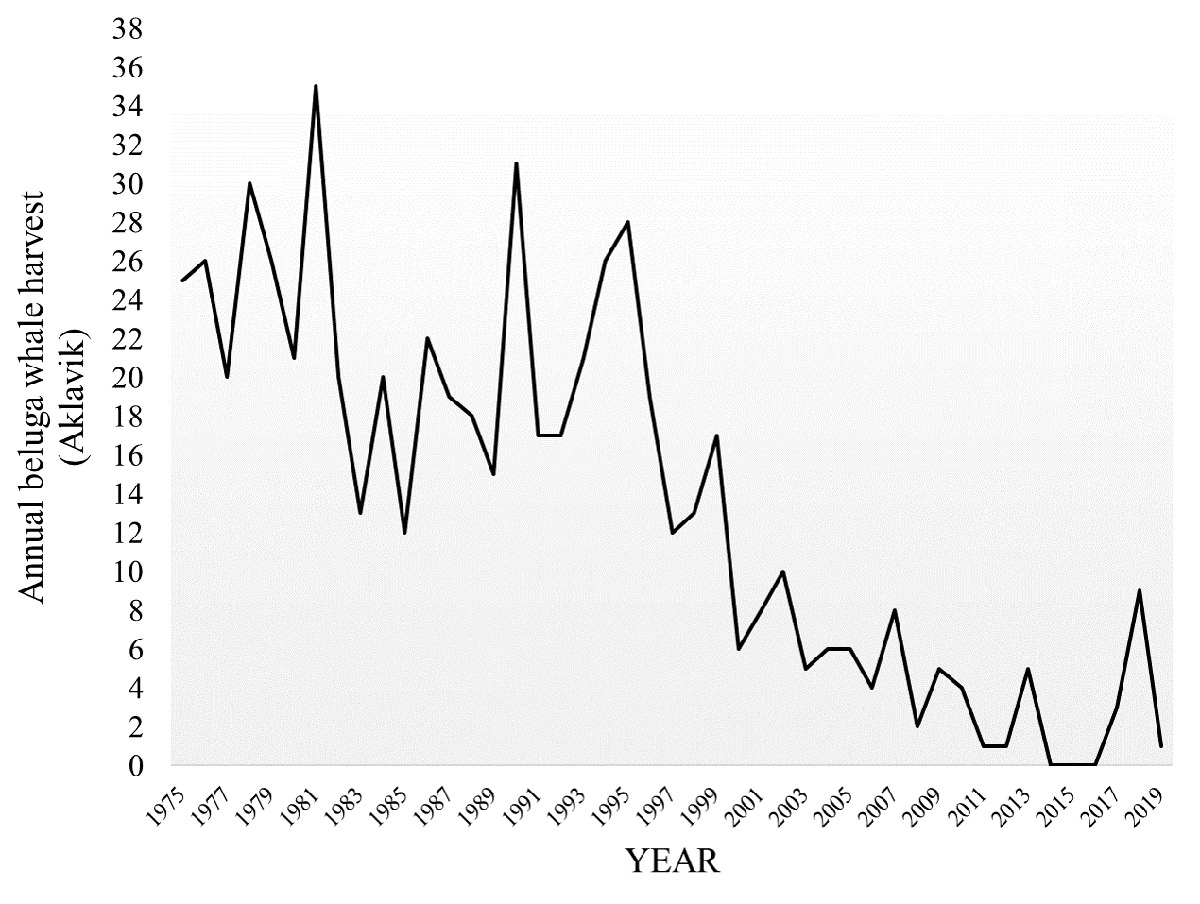
Figure 24. Aklavik’s annual beluga whale harvest from 1975-2019 (source: FJMC).
Long description
Line graph of Aklavik’s annual beluga whale harvest (number of whales from 0 to 38) (side, y-axis) from 1975 to 2013 (bottom, x-axis). A trend line for all the data shows a significant declining trend (R2 value of 0.6707). Between 1976 and 1999 harvest ranged between 12 and 35. Since 2000, harvest numbers have been below 10 per year.
References
Ardyna, M., Babin, M., Gosselin, M., Devred, E., Rainville, L., and Tremblay, J.-É. 2014. Recent Arctic Ocean sea ice loss triggers novel fall phytoplankton blooms. Geophys. Res. Lett. 41: 6207–6212. doi: 10.1002/2014GL061047.
Arnaquq-Baril, A. 2016. Angry Inuk https://www.nfb.ca/film/angry_inuk/ [Video].
Arrigo, K.R., Perovich, D.K., Pickart, R.S., Brown, Z.W., van Dijken, G.L., Lowry, K.E., Mills, M.M., Palmer, M.A., Balch, W.M., Bahr, F., Bates, N.R., Benitez-Nelson, C., Bowler, B., Brownlee, E., Ehn, J.K., Frey, K.E., Garley, R., Laney, S.R., Lubelczyk, L., Mathis, J., Matsuoka, A., Mitchell, B.G., Moore, G.W.K., Ortega-Retuerta, E., Pal, S., Polashenski, C.M., Reynolds, R.A., Scheiber, B., Sosik, H.M., Stephens, M., and Swift, J.H. 2012. Massive phytoplankton blooms under Arctic sea ice. Science 336: 1408. doi: 10.1126/science.1215065.
Barber, D.G., Hop, H., Mundy, C.J., Else, B., Dmitrenko, I.A., Tremblay, J.-É., Ehn, J.K., Assmy, P., Daase, M., Candlish,L.M., and Rysgaard, S. 2015. Selected physical, biological and biogeochemical implications of a rapidly changing arctic marginal ice zone. Prog. Oceanogr. 139: 122–150. doi: 10.1016/j.pocean.2015.09.003.
Beck, T. 1994. Alexander Charles Aviugana (1945–1994). Arctic 47: 319. doi: 10.14430/arctic1302.
Bell, R. 2009. Billy Joseph Day, whale hunter, trapper, environmentalist, September 15, 1930–July 16, 2008. Globe and Mail, Lives Lived. 2009, 21 January.
Berkes, F., Colding, J., and Folke, C. 2000. Rediscovery of traditional ecological knowledge as adaptive management. Ecol. Appl. 10: 1251–1262. doi: 10.2307/2641280.
Berkes, F., Berkes, M.K., and Fast, H. 2007. Collaborative integrated management in Canada's North: The role of local and traditional knowledge and community-based monitoring. Coast. Manage. 35: 143–162. doi: 10.1080/08920750600970487.
Blais, M., Ardyna, M., Gosselin, M., Dumont, D., Bélanger, S., Tremblay, J.-É., Gratton, Y., Marchese, C., and Poulin, M. 2017. Contrasting interannual changes in phytoplankton productivity and community structure in the coastal Canadian Arctic Ocean. Limnol. Oceanogr. 62 : 2480–2497. doi: 10.1002/lno.10581.
Bouchard, C., Geoffroy, M., LeBlanc, M., Majewski, A., Gauthier, S., Walkusz, W., Reist, J.D., and Fortier, L. 2017. Climate warming enhances polar cod recruitment, at least transiently, Prog. Oceanogr. 156: 121–129. doi: 10.1016/j.pocean.2017.06.008
Boyce, D.G., Frank, K.T., Worm, B., and Leggett, W.C. 2015. Spatial patterns and predictors of trophic control in marine ecosystems. Ecol. Lett. 18: 1001–1011. doi: 10.1111/ele.12481
Breed, G.A., Matthews, C.J.D., Marcoux, M., Higdon, J.W., LeBlanc, B., Petersen, S.D., Orr, J., Reinhart, N.R., and Ferguson, S.H. 2017. Sustained disruption of narwhal habitat use and behaviour in the presence of Arctic killer whales. Proc. Natl. Acad. Sci. USA 114: 2628–2633. doi: 10.1073/pnas.1611707114.
Burnham, K.K., Johnson, J.A., Konkel, B., and Burnham, J.L. 2012. Nesting common eider (Somateria mollissima) population quintuples in Northwest Greenland. Arctic 65: 456–464. doi: 10.14430/arctic4243.
Byers, T., Reist, J.D., and Sawatzky, C.D. 2019. Compilation and synopsis of literature on the traditional knowledge of Indigenous peoples in the Northwest Territories concerning Dolly Varden. Can. Manuscr. Rep. Fish. Aquat. Sci. 3177: vi + 63 p. Available from http://publications.gc.ca/site/eng/9.873211/publication.html [accessed 3 October 2019].
CAFF. 2013. Arctic biodiversity assessment: Status and trends in Arctic biodiversity. Conservation of Arctic Flora and Fauna, Akureyri, Iceland. 674 p. Available from https://oaarchive.arctic-council.org/handle/11374/223 [accessed 20 February 2019].
Canada. 1984. The Western Arctic claim: The Inuvialuit Final Agreement. Indian and Northern Affairs Canada, Ottawa. 259p. Available from http://webarchive.bac-lac.gc.ca:8080/wayback/20060205004956/http://www.ainc-inac.gc.ca/pr/agr/inu/wesar_e.pdf [accessed 08 February 2019].
Carmack, E.C., and Chapman, D.C. 2003. Wind‐driven shelf/basin exchange on an Arctic shelf: The joint roles of ice cover extent and shelf‐break bathymetry. Geophys. Res. Lett. 30: 1778. doi: 10.1029/2003GL017526.
Chambault, P., Albertsen, C.M., Patterson, T.A., Hanse, R.G., Tervo, O., Laidre, K.L., and Heide-Jørgensen, M.P. 2018. Sea surface temperature predicts the movements of an Arctic cetacean: The bowhead whale. Sci. Rep. 8: 9658. doi: 10.1038/s41598-018-27966-1.
Choy, E.S., Rosenberg, B., Roth, J.D., and Loseto, L.L. 2017. Inter-annual variation in environmental factors affect the prey and body condition of beluga whales in the eastern Beaufort Sea. Mar. Ecol. Prog. Ser. 579: 213–225. doi: 10.3354/meps12256.
Coupel, P., Matsuoka, A., Diana, R.P., Gosselin, M., Marie, D., Tremblay, J.-É., and Babin, M. 2015. Pigment signatures of phytoplankton communities in the Beaufort Sea. Biogeosciences 12: 991–1006. doi: 10.5194/bg-12-991-2015.
Davidson, T.A., Wetterich, S., Johansen, K.L., Grønnow, B., Windirsch, T., Jeppesen, E., Syvaranta, J., Olsen, J., González-Bergonzoni, I., Strunk, A., Larsen, N.K., Meyer, H., Søndergaard, J., Dietz, R., Eulears, I., and Mosbech, A. 2018. The history of seabird colonies and the North Water ecosystem: Contributions from palaeoecological and archaeological evidence. Ambio 47: 175–192. doi: 10.1007/s13280-018-1031-1.
Deming, J.W., Fortier, L., and Fukuchi, M. 2002. The International North Water Polynya Study (NOW): A brief overview. Deep-Sea Res. II 49: 4887–4892. doi: 10.1016/S0967-0645(02)00168-6.
DFO. 2015. Anguniaqvia Niqiqyuam area of interest: Monitoring indicators, protocols and strategies (PDF, 485 KB). DFO Can. Sci. Advis. Sec. Sci. Advis. Rep. 2015/025. Available from https://waves-vagues.dfo-mpo.gc.ca/library-bibliotheque/364882.pdf [accessed 08 February 2019].
Dorcey, A.H.J., and Hall, K.J. 1981. Setting ecological research priorities for management: The art of the impossible in the Fraser estuary. Westwater Research Centre, The University of British Columbia. Vancouver, B.C. 78p.
Dunmall, K.M., and Reist J.D. 2018. Developing a citizen science framework for the Arctic using the ‘Arctic Salmon’ initiative. In Impacts of a Changing Environment on the Dynamics of High-latitude Fish and Fisheries. Edited by F.J. Mueter, M.R. Baker, S.C. Dressel, and A.B. Hollowed. Alaska Sea Grant, University of Alaska Fairbanks. pp. 31–47. doi: 10.4027/icedhlff.2018.02.
Dunmall, K.M., Reist, J.D., Carmack, E.C., Babaluk, J.A., Heide-Jørgensen, M.P., and Docker, M.F. 2013. Pacific salmon in the Arctic: Harbingers of change. In Responses of Arctic Marine Ecosystems to Climate Change. Edited by F.J. Mueter, D.M.S. Dickson, H.P. Huntington, J.R. Irvine, E.A. Logerwell, S.A. MacLean, L.T. Quakenbush, and C. Rosa. Alaska Sea Grant, University of Alaska Fairbanks. pp. 141–163. doi: 10.4027/ramecc.2013.07.
Dunmall, K.M., Gruben, M., McNicholl, D., and Reist, J. 2017. Arctic Salmon: Linking subsistence and science to track and predict changing biodiversity of fishes in the Canadian Arctic. Integrated Regional Impact Study of the western and central Canadian Arctic (IRIS 1), December 2017 newsletter. ArcticNet. Available from http://www.arcticnet.ulaval.ca/media/iris_reports.php [accessed 08 February 2019].
Dunmall, K.M., McNicholl, D.G., and Reist, J.D. 2018. Community-based monitoring demonstrates increasing occurrences and abundances of Pacific salmon in the Canadian Arctic from 2000 to 2017. North Pacific Anadromous Fish Commission Technical Report 11: 87–90. Available from https://npafc.org/technical-report/ [accessed 08 February 2019].
Dyck, M., Campbell, M., Lee, D.S., Boulanger, J., and Hedman, D. 2017. 2016 Aerial survey of the western Hudson Bay polar bear sub-population (PDF, 3.67 MB). Final Report. Government of Nunavut, Department of Environment, Wildlife Research Section, Status Report 2017-xx, Igloolik, NU. 82 pp + 2 Supplements.
Egevang, C., Boertmann, D., Mosbech, A., and Tamstorf, M.P. 2003. Estimating colony area and population size of little auks Alle alle at Northumberland Island using aerial images. Polar Biol. 26: 8–13. doi: 10.1007/s00300-002-0448-x.
EISC. 2018. Inuvialuit Environmental Impact Screening Committee. Community Conservation Plans. Available from http://www.screeningcommittee.ca/resources/inuvialuit.html [accessed 13 September 2018].
Ferguson, S.H., Kingsley, M.C.S., and Higdon, J.W. 2012. Killer whale (Orcinus orca) predation in a multi-prey system. Popul. Ecol. 54: 31–41. doi: 10.1007/s10144-011-0284-3.
Ferguson, S.H., Young, B.G., Yurkowski, D.J., Anderson, R., Willing, C., and Nielsen, O. 2017. Demographic, ecological, and physiological responses of ringed seals to an abrupt decline in sea ice availability. PeerJ 5: e2957. doi: 10.7717/peerj.2957. PMID:28168119.
FJMC [Fisheries Joint Management Committee]. 2013. Beaufort Sea beluga management plan. 4th amended printing (PDF, 866 KB). Available from https://www.beaufortseapartnership.ca/wp-content/uploads/2015/04/Beaufort-Sea-Beluga-Management-Plan-2013.pdf [accessed 15 September 2018].
FJMC [Fisheries Joint Management Committee]. 2017. One people one plan: Inuvialuit plan for fishing on the Inuvik to Tuktoyaktuk highway (PDF, 127 KB). 5 p. Available from https://fjmc.ca/wp-content/uploads/2017/11/ITH-Community-Fishing-Plan-2017-02.pdf [accessed 15 September 2018].
Grebmeier, J.M., Cooper, L.W., Feder, H.M., and Sirenko, B.I. 2006. Ecosystem dynamics of the Pacific-influenced Northern Bering and Chukchi Seas in the Amerasian Arctic. Prog. Oceanogr. 71: 331–361. doi: 10.1016/j.pocean.2006.10.001.
Harvey, M., Starr, M., Therriault, J.C., Saucier, F., and Gosselin, M. 2006. MERICA-Nord Program: Monitoring and research in the Hudson Bay complex (PDF, 3.69 MB). AZMP Bulletin 5: 27–32. Available at https://waves-vagues.dfo-mpo.gc.ca/library-bibliotheque/365692.pdf [accessed 08 February 2019].
Harwood, L.A., Innes, S., Norton, P., and Kingsley, M.C.S. 1996. Distribution and abundance of beluga whales in the Mackenzie estuary, southeast Beaufort Sea, and west Amundsen Gulf during late July 1992. Can. J. Fish. Aquat. Sci. 53: 2262–2273. doi: 10.1139/f96-180.
Harwood, L.A., Norton, P., Day, B., and Hall, P.A. 2002. The harvest of beluga whales in Canada's western Arctic: Hunter-based monitoring of the size and composition of the catch. Arctic 55: 10–20. doi: 10.14430/arctic687.
Harwood, L.A., Smith, T.G., Georges, C., Sandstrom, S., Walkusz, W., and Divoky, G.J. 2015a. Change in the Beaufort Sea ecosystem: Diverging trends in body condition and/or production in five marine vertebrate species. Progr. Oceanogr. 136: 263–273.
doi: 10.1016/j.pocean.2015.05.003.
Harwood, L., Kingsley, M.C.S., and Pokiak, F. 2015b. Monitoring beluga harvests in the Mackenzie Delta and near Paulatuk, NT, Canada: Harvest efficiency and trend, size and sex of landed whales, and reproduction, 1970–2009. Can. Manuscr. Rep. Fish. Aquat. Sci.. 3059: vi + 32 p. Available from http://publications.gc.ca/collections/collection_2015/mpo-dfo/Fs97-4-3059-eng.pdf [accessed 08 February 2019].
Hauser, D.D.W., Laidre, K.L., Stern, H.L., Moore, S.E., Suydam, R.S., and Richard, P.R. 2017. Habitat selection by two beluga whale populations in the Chukchi and Beaufort seas. PLoS ONE 12: e0172755. doi: 10.1371/journal.pone.0172755.
Heide-Jørgensen, M.P., Strander Sinding, M.-H., Nielsen, N.H., Rosing-Asvid, A., and Hansen, R.G. 2013. The significance of the North Water polynya to arctic top predators. Ambio 42: 596–610. doi: 10.1007/s13280-012-0357-3.
Heide-Jørgensen, M.P., Sinding, M.H.S., Nielsen, N.H., Rosing-Asvid, A., and Hansen, R. G. 2016. Large numbers of marine mammals winter in the North Water polynya. Polar Biol. 39: 1605–1614. doi: 10.1007/s00300-015-1885-7.
Higdon, J.W., and Ferguson, S.H. 2009. Loss of Arctic sea ice causing punctuated change in sightings of killer whales (Orcinus orca) over the past century. Ecol. Appl. 19: 1365–1375. doi: 10.1890/07-1941.1.
Higdon, J.W., and Ferguson, S.H. 2014. Inuit recollections of a 1950s killer whale (Orcinus orca) ice entrapment in Foxe Basin, Nunavut, Canada. Aquat. Mamm 40: 9–19. doi: 10.1578/AM.40.1.2014.9.
Higdon, J.W., Westdal, K.H., and Ferguson, S.H. 2013. Distribution and abundance of killer whales (Orcinus orca) in Nunavut, Canada – an Inuit knowledge survey. J. Mar. Biol. Assoc. U.K. 94: 1293–1304. doi: 10.1017/S0025315413000921.
Hill, P.S., and DeMaster, D.P. 1999. Alaska marine mammal stock assessments, 1999. NOAA Techinical Memorandum NMFS-AFSC-110: iii + 174 p. Available from ftp://ftp.library.noaa.gov/noaa_documents.lib/NMFS/AFSC/TM_AFSC/TM_NMFS_AFSC_110.pdf [accessed 08 February 2019].
Hornby, C.A., Hoover, C., Iacozza, J., Barber, D.G., and Loseto, L.L. 2016. Spring conditions and habitat use of beluga whales (Delphinapterus leucas) during arrival to the Mackenzie River Estuary. Polar Biol. 39: 2319–2334. doi: 10.1007/s00300-016-1899-9.
Hornby, C.A., Iacozza, J., Hoover, C., Barber, D.G., and Loseto, L.L. 2017. Beluga whale Delphinapterus leucas late summer habitat use and support for foraging areas in the Canadian Beaufort Sea. Mar. Ecol. Prog. Ser. 574: 243–257. doi: 10.3354/meps12178.
Horvat, C., Jones, D.R., Iams, S., Schroeder, D., Flocco, D., and Feltham, D. 2017. The frequency and extent of sub-ice phytoplankton blooms in the Arctic Ocean. Sci. Adv. 3: e1601191. doi:10.1126/sciadv.1601191.
Hussey, N.E., Kessel, S.T., Aarestrup, K., Cooke, S.J., Cowley, P.D., Fisk, A.T., Harcourt, R.G., Holland, K.N., Iverson, S.J., Kocik, J.F., Mills Flemming, J.E., and Whoriskey, F.G. 2015. Aquatic animal telemetry: A panaromic window into the underwater world. Science 348: 1221. doi: 10.1126/science.1255642.
IPCC. 2014. Climate change 2014: Synthesis report. Contribution of Working Groups I, II and III to the Fifth Assessment Report of the Intergovernmental Panel on Climate Change [Core Writing Team, R.K. Pachauri and L.A. Meyer (eds.)]. IPCC, Geneva, Switzerland. 151 p. Available from https://www.ipcc.ch/report/ar5/syr/ [accessed 08 February 2019].
Irvine, J.R., and Riddell, B.E. 2007. Salmon as status indicators for North Pacific ecosystems. N. Pac. Anadr. Fish Comm. Bull. 4: 285–287. Available from https://npafc.org/wp-content/uploads/2017/09/bulletin4.pdf [accessed 08 February 2019].
Jeppesen, E., Appelt, M., Hastrup, K., Grønnow, B., Mosbech, A., Smol, J.P., and Davidson, T.A. 2018. Living in an oasis: Rapid transformations, resilience, and resistance in the North Water Area societies and ecosystems. Ambio 47: 296–309. doi: 10.1007/s13280-018-1034-y.
Kovacs, K., and Michel, C. 2011. Biological impacts of changes to sea ice in the Arctic. In Snow, Water, Ice and Permafrost in the Arctic (SWIPA): Climate Change and the Cryosphere. Arctic Monitoring and Assessment Programme (AMAP), Oslo, Norway. pp. 9-32–9-51. Available from https://www.amap.no/documents/doc/snow-water-ice-and-permafrost-in-the-arctic-swipa-climate-change-and-the-cryosphere/743 [accessed 08 February 2019].
Laidlaw, D. 2015. Challenges in using Aboriginal traditional knowledge in the courts. In A Symposium on Environment in the Courtroom: Evidentiary Issues in Environmental Prosecutions and Hearings, March 6-7, 2015. University of Calgary.
Lewis, A.E., Hammill, M.O., Power, M., Doidge, D.W., and Lesage, V. 2009. Movement and aggregation of eastern Hudson Bay beluga whales (Delphinapterus leucas): A comparison of patterns found through satellite telemetry and Nunavik traditional ecological knowledge. Arctic 62: 13–24. doi: 10.14430/arctic109.
Loseto, L.L., Stern, G.A., Connelly, T.L., Deibel, D., Gemmill, B., Prokopowicz, A., Fortier, L., and Ferguson, S.H. 2009. Summer diet of beluga whales inferred by fatty acid analysis of the eastern Beaufort Sea food web. J. Exp. Mar. Biol. Ecol. 374: 12–18. doi: 10.1016/j.jembe.2009.03.015.
Loseto, L., Wazny, T., Cleator, H., Ayles, B., Cobb, D., Harwood, L., Michel, C., Nielsen, O., Paulic, J., Postma, L., Ramlal, P., Richard, P., Ross, P.S., Solomon, S., Walkusz, W., Weilgart, L., and Williams, B. 2010. Information in Support of Indicator Selection for Monitoring the Tarium Niryutait Marine Protected Area (TN MPA). DFO Can. Sci. Advis. Sec. Res. Doc. 2010/094. iv + 42 p. Available from www.dfo-mpo.gc.ca/csas-sccs/Publications/ResDocs-DocRech/2010/2010_094-eng.html [accessed 08 February 2019].
Loseto, L.L., Brewster, J.D., Ostertag, S.K., Snow, K., MacPhee, S.A., McNicholl, D.G., Choy, E.S., Giraldo, C., and Hornby, C.A. 2018a. Diet and feeding observations from an unusual beluga harvest in 2014 near Ulukhaktok, Northwest Territories, Canada. Arctic Science 4: 421–431. doi: 10.1139/as-2017-0046.
Loseto, L.L., Lam, J., and Iacozza, J. (eds.). 2018b. Beluga Summit: knowledge sharing of the eastern Beaufort Sea beluga whale. Arctic Science 4: i–iv. doi: 10.1139/as-2018-0011.
Loseto, L.L., Hoover, C., Ostertag, S., Whalen, D., Pearced, T., Paulic, J., Iacozza, J., and MacPhee, S. 2018c. Beluga whales (Delphinapterus leucas), environmental change and marine protected areas in the Western Canadian Arctic. Estuar. Coast. Shelf Sci. 212: 128–137. doi: 10.1016/j.ecss.2018.05.026.
Lunn, N. J., Servanty, S., Regehr, E.V., Converse, S.J., Richardson, E. and Stirling, I. 2016. Demography of an apex predator at the edge of its range: Impacts of changing sea ice on polar bears in Hudson Bay. Ecol. Appl. 26: 1302–1320. doi: 10.1890/15-1256.
Marchese, C., Albouy, C., Tremblay, J.-É.., Dumont, D., D’Ortenzio, F., Vissault, S., and Bélanger, S. 2017. Changes in phytoplankton bloom phenology over the North Water (NOW) polynya: A response to changing environmental conditions. Polar Biol. 40: 1721–1737. doi: 10.1007/s00300-017-2095-2.
Matthews, C.J.D., Luque, S.P., Petersen, S.D., Andrews, R.D., and Ferguson, S.H. 2011. Satellite tracking of a killer whale (Orcinus orca) in the eastern Canadian Arctic documents ice avoidance and rapid, long-distance movement into the North Atlantic. Polar Biol. 34:1091–1096. doi: 10.1007/s00300-010-0958-x.
Matthews, C.J.D., Raverty, S.A., Noren, D.P., Arragutainaq, L., and Ferguson, S.H. 2019. Ice entrapment mortality may slow expanding presence of Arctic killer whales. Polar Biol. 42: 639–644. doi: 10.1007/s00300-018-02447-3.
McGhee, R. 1988. Beluga hunters: An archaeological reconstruction of the history and culture of the Mackenzie Delta Kittegaryumiut. Institute of Social and Economic Research, Memorial University of Newfoundland, St. John’s. 124 p.
McNicholl, D., and Dunmall, K. 2018a. Sachs Harbour coastal baseline July 2018. Available from https://www.facebook.com/arcticsalmon/photos/a.140681756049965/1850298851754905/?type=3&theater [accessed 08 February 2019].
McNicholl, D., and Dunmall, K. 2018b. Darnley Bay Coastal fish survey - July 3rd-18th, 2018. Available from https://tinyurl.com/Darnley-Bay-2018 [accessed 08 February 2019].
McNicholl, D.G., Johnson, J.D., and Reist, J.D. 2017. Darnley Bay nearshore survey: Synthesis of 2012 and 2014–2016 field programs. Can. Tech. Rep. Fish. Aquat. Sci. 3229: ix + 101 p. Available from http://publications.gc.ca/collections/collection_2017_mpo-dfo/Fs97-6-3229-eng.pdf [accessed 08 February 2019].
Merkel, F., Labansen, A.L., Boertmann, D., Mosbech, A., Egevang, C., Falk, K., Linnebjerg, J.F., Frederiksen, M., and Kampp, K. 2014. Declining trends in the majority of Greenland’s thick-billed murre (Uria lomvia) colonies 1981–2011. Polar Biol. 37: 1061–1071. doi: 10.1007/s00300-014-1500-3.
Michel, C., Hamilton, J., Hansen, E., Barber, D., Reigstad, M., Iacozza, J., Seuthe, L., and Niemi, A. 2015. Arctic Ocean outflow shelves in the changing Arctic: A review and perspectives. Prog. Oceanogr. 139: 66–88. doi: 10.1016/jpocean.2015.08.007.
Moore, S.E., and Huntington, H.P. 2008. Arctic marine mammals and climate change: Impacts and resilience. Ecol. Appl. 18: S157–S165. doi: 10.1890/06-0571.1.
Mueter, F., Nahrgang, J., Nelson, R.J., and Berge, J. 2016. The ecology of gadid fishes in the circumpolar Arctic with a special emphasis on the polar cod (Boreogadus saida). Polar Biol. 39: 961–967. doi: 10.1007/s00300-016-1965-3.
Mundy, C.J., Gosselin, M., Gratton, Y., Brown, K., Galindo, V., Campbell, K., Levasseur, M., Barber, D., Papkyriakou, T., and Bélanger, S. 2014. Role of environmental factors on phytoplankton bloom initiation under landfast sea ice in Resolute Passage, Canada. Mar. Ecol. Prog. Ser. 497: 39–49. doi: 10.3354/meps10587.
Nielsen, J.L., Ruggerone, G.T., and Zimmerman, C.E. 2013. Adaptive strategies and life history characteristics in a warming climate: Salmon in the Arctic? Environ. Biol. Fish. 96: 1187–1226. doi: 10.1007/s10641-012-0082-6.
Obbard, M.E., Cattet, M.R.L., Howe, E.J., Middel, K.R., Newton, E.J., Kolenosky, G.B., Abraham, K.F., and Greenwood, C.J. 2016. Trends in body condition in polar bears (Ursus maritimus) from the southern Hudson Bay subpopulation in relation to changes in sea ice. Arctic Science 2: 15–32. doi: 10.1139/as-2015-0027.
Obbard, M.E., Stapleton, S., Szor, G., Middel, K.R., Jutras, C., and Dyck, M. 2018. Re-assessing abundance of southern Hudson Bay polar bears by aerial survey: Effects of climate change at the southern edge of the range. Arctic Science 4: 634–655. doi: 10.1139/as-2018-0004.
Ostertag, S., Loseto, L., Snow, K., Lam, J., Hynes, K., and Gillman, V. 2018. “That’s how we know they’re healthy”: The inclusion of Indigenous Knowledge in beluga health monitoring in the Inuvialuit Settlement Region. Arctic Science 4: 292–320. doi: 10.1139/as-2017-0050.
Palmer, M.A., Saenz, B.T., and Arrigo, K.A. 2014. Impacts of sea ice retreat, thinning, and melt-pond proliferation on the summer phytoplankton bloom in the Chukchi Sea, Arctic Ocean. Deep Sea Res. II 105: 85–104. doi: 10.1016/j.dsr2.2014.03.016.
Pikialasorsuaq Commission. 2017. People of the ice bridge: The future of the Pikialasorsuaq. Report of the Pikialasorsuaq Commission. November 2017. 119 p. Available from http://www.pikialasorsuaq.org/en/Resources/Reports [accessed 09 February 2019].
Polar Knowledge Canada. 2017. Advancing polar science and collaboration: Polar Knowledge Canada report 2015–2017 (PDF, 3.19 MB). Polar Knowledge Canada, Ottawa, ON. 28 p.
Reist, J.D., Wrona, F.J., Prowse, T.D., Power, M., Dempson, J.B., King, J.R., and Beamish, R.J. 2006a. An overview of effects of climate change on selected Arctic freshwater and anadromous fishes. Ambio 35: 381–387. doi: 10.1579/0044-7447(2006)35%5B381:AOOEOC%5D2.0.CO;2.
Reist, J.D., Wrona, F.J., Prowse, T.D., Power, M., Dempson, J.B., Beamish, R.J., King, J.R., Carmichael, T.J., and Sawatzky, C.D. 2006b. General effects of climate change on Arctic fishes and fish populations. Ambio 35: 370–380. doi: 10.1579/0044-7447(2006)35[370:GEOCCO]2.0.CO;2.
Riedlinger, D., and Berkes, F. 2001. Contributions of traditional knowledge to understanding climate change in the Canadian Arctic. Polar Rec. 37: 315–328. doi: 10.1017/S0032247400017058.
RSEA. 2018. Beaufort Sea Regional Strategic Environmental Assessment.
https://rsea.inuvialuit.com.
Sciullo, L., Thiemann, G.W., and Lunn, N.J. 2016. Comparative assessment of metrics for monitoring the body condition of polar bears in western Hudson Bay. J. Zool. 300: 45–58. doi: 10.1111/jzo.12354.
Stevenson, M.G. 2004. Decolonizing co-management in northern Canada. Cultural Survival Quarterly 28-1: 68.
Stirling, I. 1997. The importance of polynyas, ice edges, and leads to marine mammals and birds. J. Mar. Syst. 10: 9–21. doi: 10.1016/S0924-7963(96)00054-1.
Stirling, I., Lunn, N. J., and Iacozza, J. 1999. Long-term trends in the population ecology of polar bears in Western Hudson Bay in relation to climatic change. Arctic 52: 294–306. doi: 10.14430/arctic935.
Syvitski, J.P.M., LeBlanc, K.W.G., and Cranston, R.E. 1990. The flux and preservation of organic carbon in Baffin Island fjords. Geol. Soc. London Spec. Publ. 53: 177–199. doi: 10.1144/GSL.SP.1990.053.01.10.
Towns, L., Derocher, A.E., Stirling, I., Lunn, N.J., and Hedman, D. 2009. Spatial and temporal patterns of problem bears in Churchill, Manitoba. Polar Biol. 32: 1529–1537. doi: 10.1007/s00300-009-0653-y.
Tremblay, J.-É., Michel, C., Hobson, K.A., Gosselin, M., and Price, N.M. 2006a. Bloom dynamics in early-opening waters of the Arctic Ocean. Limnol. Oceanogr. 51: 900–912. doi: 10.4319/lo.2006.51.2.0900.
Tremblay, J-É., Hattori, H., Michel, C., Ringuette, M., Mei, Z.-P., Lovejoy, C., Fortier, L., Hobson, K.A., Amiel, D., and Cochran, K. 2006b. Trophic structure and pathways of biogenic carbon flow in the eastern North Water Polynya. Prog. Oceanogr. 71: 402–425. doi: 10.1016/j.pocean.2006.10.006.
Tremblay, M., Frugal, C., Larrivee, C., Annanack, T., Tookalook, P., Qiisik, M., Angiyou, E., Swappie, N., Savard, J., and Barrett, M. 2008. Climate change in northern Quebec: Adaptation strategies from community-based research. Arctic 61: 27–34. doi: 10.14430/arctic99.
Watt, C.A., Orr, J., and Ferguson, S.H. 2016. A shift in foraging behaviour of beluga whales Delphinapterus leucas from the threatened Cumberland Sound population may reflect a changing Arctic food web. Endanger. Species Res. 31: 259–270. doi: 10.3354/esr00768.
Watt, C.A., Orr, J., and Ferguson, S.H. 2017. Spatial distribution of narwhal (Monodon monoceros) diving for Canadian populations helps identify important seasonal foraging areas. Can. J. Zool. 95: 41–50. doi: 10.1139/cjz-2016-0178.
Waugh, D., Pearce, T, Ostertag, S.K., Pokiak, V., Collings, P., and Loseto, L. 2018. Inuvialuit traditional ecological knowledge of beluga whale (Delphinapterus leucas) under changing climatic conditions in Tuktoyaktuk, NT. Arctic Science 4: 242–258. doi: 10.1139/as-2017-0034.
Westdal, K.H., Davies, J., MacPherson, A., Orr, J., and Ferguson, S.H. 2016a. Behavioural changes in belugas (Delphinapterus leucas) during a killer whale (Orcinus orca) attack in Southwest Hudson Bay. Can. Field-Nat. 130: 315–319. doi: 10.22621/cfn.v130i4.1925.
Westdal, K.H., Higdon, J.W., and Ferguson, S.H. 2016b. Review of killer whales (Orcinus orca) ice entrapments and ice-related mortality events in the northern hemisphere. Polar Biol. 40: 1467–1473. doi: 10.1007/s00300-016-2019-6.
White, G. 2006. Cultures in collision: Traditional knowledge and Euro-Canadian governance processes in native land-claim boards. Arctic 59: 401–414. doi: 10.14430/arctic289.
Worden, E. 2018. “Everything is changing so much”: Community Perspectives on the Declining Beluga Whale Harvest in Aklavik, NT. Thesis (M.Sc.) University of Manitoba, Winnipeg, MB. viii + 188 p. Available from https://mspace.lib.umanitoba.ca/xmlui/handle/1993/33654 [accessed 13 February 2019].
Yoon, S., Watanabe, E., Hiromichi, U., and Kishi, M.J. 2015. Potential habitat for chum salmon (Oncorhynchus keta) in the Western Arctic based on a bioenergetics model coupled with a three-dimensional lower trophic ecosystem model. Prog. Oceanogr. 131: 146–158. doi: 10.1016/j.pocean.2014.12.009.
Young, B.G., Higdon, J.W., and Ferguson, S.H. 2011. Killer whale (Orcinus orca) photo-identification in the eastern Canadian Arctic. Polar Res. 30: 7203. doi: 10.3402/polar.v30i0.7203.
Yurkowski, D.J., Auger‐Méthé, M., Mallory, M.L., Wong, S.N.P., Gilchrist, G., Derocher, A.E., Richardson, E., Lunn, N.J., Hussey, N.E., Marcoux, M., Togunov, R.R., Fisk, A.T., Harwood, L.A., Dietz, R., Rosing-Asvid, A., Born, E.W., Mosbech, A., Fort, J., Grémillet, D., Loseto, L., Richard, P.R., Iacozza, J., Jean-Gagnon, F., Brown, T.M., Westdal, K.H., Orr, J., LeBlanc, B., Hedges, K.J., Treble, M.A., Kessel, S.T., Blanchfield, P.J., Davis, S., Maftei, M., Spencer, N., McFarlane-Tranquilla, L., Montevecchi, W.A., Bartzen, B., Dickson, L., Anderson, C., and Ferguson, S.H. 2019. Abundance and species diversity hotspots of tracked marine predators across the North American Arctic. Divers. Distrib. 25: 328–345. doi: 10.1111/ddi.12860.
- Date modified: