Marteilia sydneyi of Oysters
On this page
Category
Category 3 (Host Not in Canada)
Common, generally accepted names of the organism or disease agent
QX (Queensland unknown) disease.
Scientific name or taxonomic affiliation
Marteilia sydneyi assigned to the phylum Paramyxea in the past (Berth et al. 2000, 2004) was transferred to the phylum Cercozoa, supergroup Rhizaria, group Ascetosporea and order Paramyxida (Cavalier-Smith and Chao 2003, Feist et al. 2009, Ward et al. 2016). The "haplosporidian" reported in Sydney rock oysters, Saccostrea (=Crassostrea) glomerata (=commercialis), from Moreton Bay, Queensland, Australia by Wolf (1972) was named M. sydneyi by Perkins and Wolf (1976).
Geographic distribution
In coastal estuaries of southern Queensland and New South Wales, Australia (Nell 1993, Adlard and Ernst 1995, Kleeman et al. 2004, Adlard and Wesche 2005), representing a range of approximately 950 km (Guo and Ford 2016). Infection with M. sydneyi was detected in 1 of 117 Saccostrea glomerata from Dampier Archipelago, Western Australia (Hine and Thorne 2000) and in 1 of 411 S. glomerata from the same area in 1995 (Jones and Creeper 2006). Hine and Thorne (2000) also detected a Marteilia sp. in 1 of 26 Saccostrea cucullata from the Montebello Islands, Western Australia. They indicated that this parasite resembled Marteilia lengehi from S. cucullata in the Persian Gulf that was described by Comps (1976). A Marteilia-like parasite was reported in 2 of 29 Saccostrea cucullata (=forskali) in Thailand (Taveekijakarn et al. 2002, 2008). Carrasco et al. (2015) suggested that this parasite is morphologically similar to M. sydneyi.
Host species
Marteili sydneyi was described from Saccostrea (=Crassostrea) glomerata (=commercialis) and possibly also occurs in Saccostrea echinata (=Crassostrea =Striostrea mytiloides) and Saccostrea cucullata (=forskali) (Wolf 1977, Kleeman et al. 2002b, Taveekijakarn et al. 2008). Similar protistans were reported from Ostrea angasi and clams including the giant clam Tridacna maxima. Apparently, Crassostrea gigas does not suffer from QX disease caused by infection with Marteilia sydneyi (Nell 2001). Using molecular tools, Adlard and Nolan (2009, 2015) detected M. sydneyi in the digestive tract epithelium of the polycheate worm Nephtys australiensis (Aranguren and Figueras 2016).
Impact on the host
Typically, infected oysters are in poor condition with their gonad completely resorbed. Apparently, M. sydneyi may kill up to 80% of infected oysters with up to 100% prevalence (Wolf 1977, Hine 1996). Nell (1993, 2001, 2002) indicated that wherever M. sydneyi has been detected, the oyster industry has severely declined. Although the decline was slow (taking 30 years) in some areas of northern New South Wales, in the Georges River, Sydney, the industry totally collapsed in 2001 within seven years of M. sydneyi first being detected at that area. The disease is also a threat to other S. glomerata production areas in Australia (Bezemer et al. 2006). Massive invasion of the digestive gland epithelial cells by M. sydneyi leads to complete disorganization of the infected tissue. Death results from starvation and may occur in less than 60 days after initial infection (Wolf 1977). Disease development is favoured by lower salinities (Meyers 2006). Peters and Raftos (2003) indicated that phenoloxidase activity was significantly suppressed in oysters associated with QX disease and that inhibition of the prophenoloxidase cascade may facilitate lethal infection by M. sydneyi. However, the presence of M. sydneyi in oysters does not always result in the development of QX disease which is more closely associated with the sporulation of the parasite (Simonian et al. 2009a). Haemocytes of S. glomerata can recognise and phagocytose M. sydneyi, where granules fused with phagosome membranes, the pH of phagosomes decreased, phenoloxidase deposition occurred, and ingested and melanised M. sydneyi were detected in vivo among haemocytes from infected oysters (Kuchel et al. 2010). Butt and Raftos (2008) indicated that phenoloxidase activity led to the complete melanisation of phagosomes and suggested that phagocytosis and cellular melanisation are critical defensive responses of S. glomerata infected with M. sydneyi. Also, Butt and Raftos (2007) suggested that the presence of some transient environmental stressor may affect phenoloxidase activity during a key period of infection and thus increase the susceptibility of oysters to disease. Green and Barnes (2010) employing DNA analysis techniques found that the microbiota of the digestive gland of S. glomerata was changed by infection with M. sydneyi, becoming dominated by a Rickettsiales-like prokaryote, and generally less diverse.
Oysters are most susceptible to disease caused by M. sydneyi during the warm months (Guo and Ford 2016), although Wolf (1979) reported epidemics in all seasons in northern estuaries of New South Wales, Australia. Lester (1986), Anderson et al. (1994a), Wesche (1995) and Adlard (1997) conducted transplant experiments and determined that oysters may be exposed to infection over a very short interval (possibly only two weeks per year). However, Rubio et al. (2013) reported that the onset of the infection window of M. sydneyi in S. glomerata can last between 8 and 18 weeks and often follows a rapid decrease in water salinity, although they were not able to determine the exact environmental conditions that triggered the onset. Nevertheless, temperature was shown to be a key parameter in the life cycle of M. sydneyi (Rubio et al. 2013). Once infection was acquired, warm temperatures favour parasite development and host mortalities are greatest at the end of summer. At lower temperatures, retardation of host mortality and suppression of parasite development occurs. In some cases, infected oysters may survive the summer months and over winter with the disease, however subsequent elevated temperatures usually induce mortality. At times, M. sydneyi has caused over 90% mortality on oyster farms in northern New South Wales and southern Queensland (Lester 1986, Anderson et al. 1994b. Adlard 1997). No mortalities were associated with the detection of a M. sydneyi-like parasite in Saccostrea cucullata (=forskali) from two sites at the upper part of the Gulf of Thailand (Taveekijakarn et al. 2008).
There was no apparent correlation with epizootics (outbreaks) of M. sydneyi and fluctuations in pH, salinity and temperature (Anderson et al. 1994a;, Wesche 1995). The lack of correlation between pH and M. sydneyi infection of S. glomerata (Anderson et al. 1994a), may indicating that pH is more influential on the oyster host, rather than influencing the protozoan parasite itself (King et al. 2019). Adlard and Wesche (2005) determined that the presence of M. sydneyi in estuaries where disease has not been recorded has emphasised the likely role played by the dynamics of the parasite's lifecycle together with host immune defense and likely environmental factors as regulators of disease outbreaks in S. glomerata. Specifically, immunological studies by Peters and Raftos (2003) and Butt and Raftos (2007) have shown the immune system of S. glomerata to be compromised by the presence of some transient environmental stressor in estuaries enzootic for QX-disease. Also, historical data suggests that land-use practices have resulted in sedimentation of Australian rivers and estuaries resulting in the increased prevalence of M. sydneyi due to water quality decline and the increased abundance of possible alternative hosts in the habitat utilized by oysters (Diggles 2013). Thus, environmental degradation is probably a contributing factor in QX disease (Carrasco et al. 2015).
The developmental stages of M. sydneyi in S. glomerata were identified and illustrated by Kleeman et al. (2002a). The initial infective stage of M. sydneyi enters S. glomerata through the palps and gills where extrasporogonic proliferation (i.e., proliferation that does not result in the formation of spores) occurs in the epithelium. A daughter cell within a vacuole in the cytoplasm of the uninucleate stem cell divides by binary fission to produce four daughter cells within the enlarged stem cell. Internal cleavage results in the formation of an uninucleate cell within each daughter cell. The stem cell degenerates to release the daughter cells, which become new stem cells. Following proliferation, stem cells each containing a daughter cell are liberated into surrounding connective tissue and haemolymph spaces to form a transient systemic stage. Following systemic dissemination, the parasites infiltrate the digestive gland, penetrate the basal membrane of the tubules and become established as nurse cells at the base of the epithelial cells in the digestive gland tubules. The nurse cell elongates and forms pseudopodial extensions that protrude along the basal membrane. Daughter cells formed within the nurse cells divide and are thus spread along the basal membrane to establish new infections between adjacent epithelial cells until all available sites within the digestive tubule are occupied. In mature infections, the nurse cells degrade and each daughter cell becomes the primary cell described by Perkins and Wolf (1976). The primary cell internally cleaves a secondary cell that divides to form a sporangiosorus (sporont primordia) containing 8 to 16 sporonts (sporangia) marking the initiation of sporulation. The sporulation process involves internal cleavage to produce two tertiary precursor cells, each of which develop into tricellular spores, within each sporont within a sporangiosorus (Perkins and Wolf 1976). Mature spores are shed into the tubule lumen for evacuation from the oyster and infected oysters shed large numbers (almost all) of the spores before oyster death (Carrasco et al. 2015, King et al. 2019). However, a few S. glomerata have been observed to eliminate low levels of M. sydneyi infection by shedding the parasite and undergoing full recovery (Roubal et al. 1989). Nevertheless, spores of M. sydneyi were not infective to S. glomerata.
The rest of the life cycle is unknown but Roubal et al. (1989) implicated filter-feeding or detritivorous invertebrates rather than scavenging invertebrates or fish in the life cycle. Adlard and Nolan (2009, 2015) sampled the macrobenthos of the Hawkesbury River, New South Wales (Australia) where M. sydneyi is known to occur and examined polycheate worms using qPCR, in-situ hybridization (ISH) and histology. Seventy six of 1247 samples of at least eight species of polycheate worms were PCR positive for M. sydneyi. Of 116 specimens from five of the PCR positive species tested by ISH, only two specimens of Nephtys australiensis were positive for M. sydneyi. Nevertheless, in these two specimens of N. australiensis, they found two different developmental stages (30 by 10 µm in dimension) of M. sydneyi associated with or adhered to the intestinal epithelium of the polychaete worm. A 'primordial' cell with a well-defined nucleus and little differentiation in the cytoplasm and a 'plasmodial' cell that showed an apparent multi-nucleated syncytial structure. They speculated that N. australiensis may be critical for the maturation and transmission of M. sydneyi. (Adlard and Nolan 2015). However, as indicated by Carrasco et al. (2015), it is yet to be determined if N. australiensis may act as a reservoir for M. sydneyi, or as the secondary host supporting the next stage in the life cycle of M. sydneyi, or is a ''dead end'' infection.
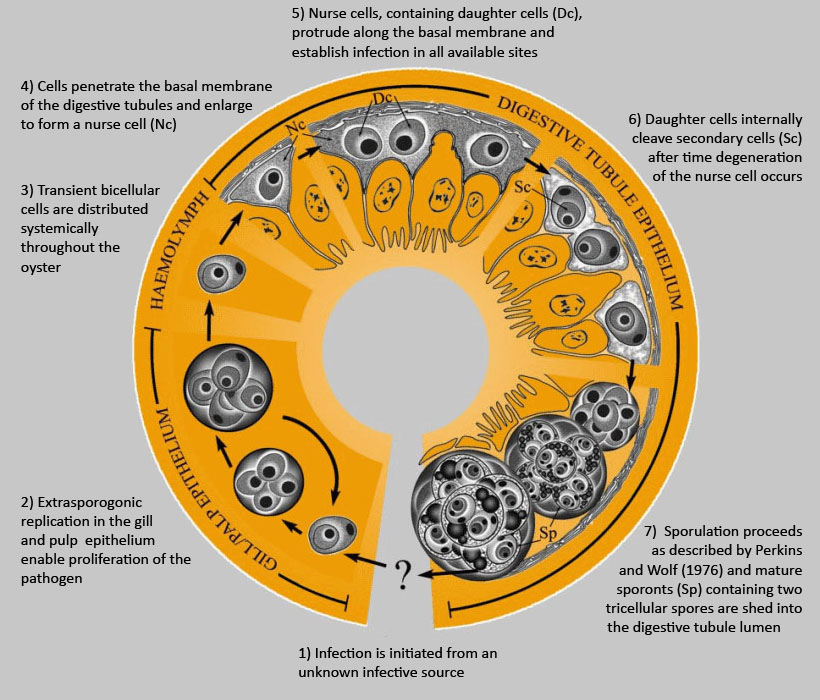
Figure 1. Hypothetical development of Marteilia sydneyi in the Sydney rock oyster, Saccostrea glomerata. Image provided by S.N. Kleeman, Biosecurity Australia.
Hypothetically, the development of Marteilia sydneyi in the Sydney rock oyster would happen in seven stages:
- infection is initiated from an unknown infective source,
Gill/Pale Epithelium
- extrasporogonic replication in the gill and pulp epithelium enable proliferation of the pathogen,
Haemolymph
- transient bicellular cells are distributed systemically throughout the oyster,
Digestive Tubule Epithelium
- cells penetrate the basal membrane of the digestive tubules and enlarge to form a nurse cell,
- nurse cells, containing daughter cells (Dc), protrude along the basal membrane and establish infection in all available sites,
- daughter cells internally cleave secondary cells (Sc) after time degeneration of the nurse cell occurs, and
- sporulation proceeds as described by Perkins and Wolf (1976) and mature sporonts (Sp) containing two tricellular spores are shed into the digestive tubule lumen.
Diagnostic techniques
Gross Observations
The pale yellow-brown colour of the digestive gland contrasts with the deep green of healthy oysters. The body is greatly shrunken and tissues are translucent due to the complete resorption of the gonad.
Wet Mounts
Refractile bodies in sporonts in wet smears of digestive gland (hepatopancrease) provide a simple and rapid diagnosis when sporonts are present in infected oysters.
Figures 2 and 3. Unstained wet mount squashes of the digestive gland of Saccostrea glomerata infected with mature stages of Marteilia sydneyi. Images provided by S.N. Kleeman, Biosecurity Australia.
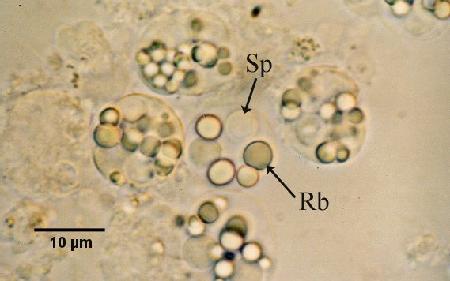
Figure 2. Sporonts of M. sydneyi containing refractile bodies (Rb) and spores (Sp).
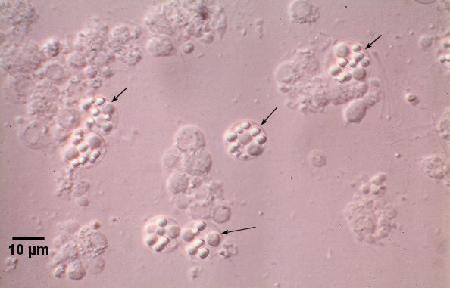
Figure 3. Sporonts of M. sydneyi (arrows) viewed under interference contrast optics.
Tissue Imprints
Dried imprints of the digestive gland (hepatopancrease) on glass slides stained with Wright, Wright-Giemsa or equivalent stain (e.g. Hemacolor, Merck; Diff-QuiK, Baxter) enables the rapid detection of all developmental stages including presporulation stages not detectable in wet mount preparations within a few days of initial infection (Kleeman and Adlard 2000). Scan slides for the presence of M. sydneyi, at 200x and 400x magnification. The oil emersion objective (1000x magnification) may be required for definitive identification of early stages of infection in the digestive gland (i.e. daughter or primary cell stages). In all but very early stages of M. sydneyi incursion into the digestive gland, the infection was sufficiently homogeneous that sub-sampling of tissue for diagnosis did not have a significant negative impact on the ability of diagnostic tests to detect the disease (Adlard and Wesche 2005). A comparison between tissue imprints (cytology) and histology showed that cytology (96.88% infected) had a greater sensitivity than histology (86.11% infected) (Adlard and Wesche 2005).
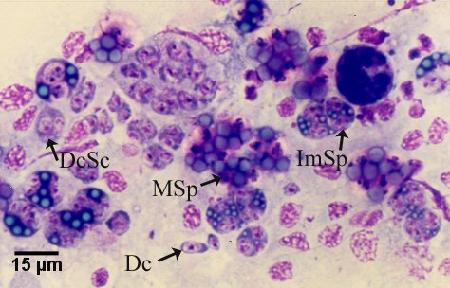
Figure 4. Hemacolor (Merck) stained tissue imprint of the digestive gland of Saccostrea glomerata infected with Marteilia sydneyi, showing various life cycle stages including daughter cells (Dc), daughter cells containing secondary cells (DcSc), immature sporonts (ImSp), and mature sporonts (MSp). Note that the various stages observed are often ruptured from their enclosing cells (i.e. the nurse cells or sporangiosori). Image provided by S.N. Kleeman, Biosecurity Australia.
Histology
Examine cross-sections of the digestive gland for the presence of Marteilia in the digestive gland epithelial cells. Marteilia sydneyi can be differentiated from Marteilia refringens by 1) the lack of striated inclusions in the sporont primordia, 2) the formation of eight to sixteen sporonts (sporangial primordia, sporangia) in each sporangiosorus (plasmodium), instead of eight, 3) the occurrence of two, rather than four, spores in each sporont (sporangium), and 4) the heavy layer of concentric membranes surrounding mature spores, in comparison to the lack of such a covering around M. refringens spores. Detection of infection has been facilitated by recent descriptions of the early developmental stages and site of infection (Kleeman et al. 2001, 2002a,c) and haemocyte infiltration throughout the tissue is common in pre-sporulating infections (Adlard and Wesche 2005). However, detection of the initial infections is facilitated by the application of DNA probes via in situ hybridisation (see Figs. 8 and 9, Fig. 10 and details pertaining to the DNA Probes below, Kleeman et al. 2002b, Adlard and Wesche 2005).
Figures 5 to 7. Extrasporogonic replication of Marteilia sydneyi in the gill and palp epithelium of Saccostrea glomerata following the initiation of infection from an unknown source. Haematoxylin and eosin stain. Images provided by S.N. Kleeman, Biosecurity Australia.
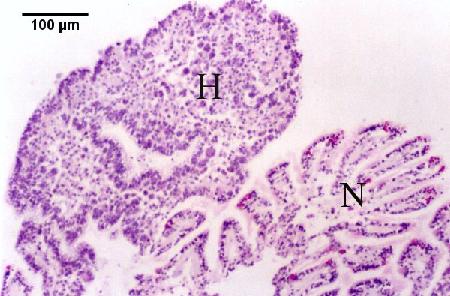
Figure 5. Oyster reaction consisting of epithelial and connective tissue hyperplasia (H) and fusion of filaments due to the presence of numerous extrasporogonic stages in the epithelium of the gills in contrast to relatively normal looking gill tissue (N).
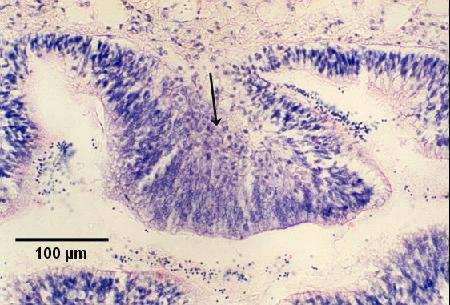
Figure 6. Replicating stages in the palp epithelium. Note the hypertrophy of the epithelial cells in the presence of proliferating parasites (arrow) in infected areas.
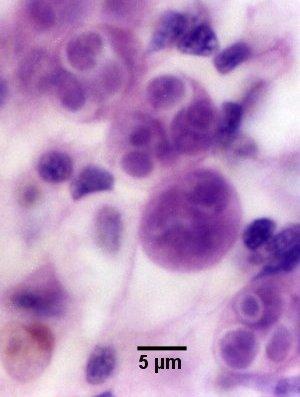
Figure 7. Higher magnification of extrasporogonic stages in the epithelium of the gills (see phase 2 in the life cycle diagram).
Figures 8 and 9. Tissue sections through the digestive gland of Saccostrea glomerata demonstrating an early stage of disease development. Images provided by S.N. Kleeman, Biosecurity Australia.
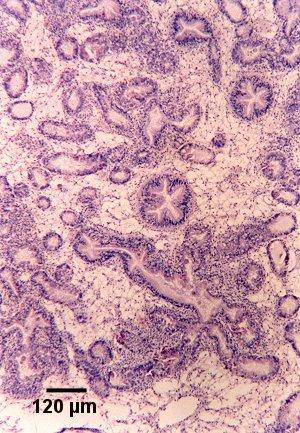
Figure 8. Section showing haemocytic infiltration of the connective tissue surrounding infected digestive gland tubules. Haematoxylin and eosin stain.
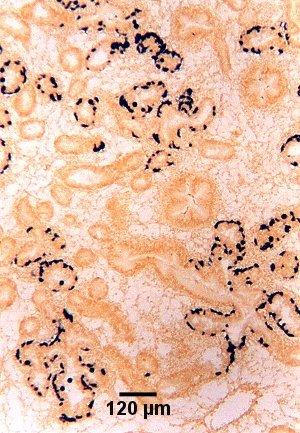
Figure 9. Tissue section cut adjacent to that shown in Fig. 8 and showing the location of presporulating nurse cell stages (stained black) in digestive gland tubule epithelia as detected by in situ hybridisation using the Smart 2 probe as described below.
Figures 10 to 15. Presporulating stages of Marteilia sydneyi in the digestive gland tubules of Saccostrea glomerata. Haematoxylin and eosin stain except for Fig. 10 which was coloured by in situ hybridisation using the Smart 2 probe as described below. Images provided by S.N. Kleeman, Biosecurity Australia.
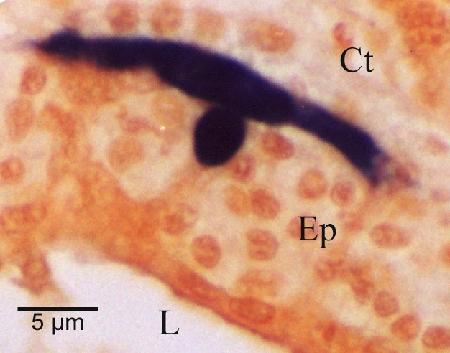
Figure 10. Nurse cell (stained black by in situ hybridisation) demonstrating the extent of the pseudopodial extensions along the basal membrane of the digestive tubule epithelium (Ep). This feature is not evident with haematoxylin and eosin staining. Other labeled features are the connective tissue (Ct) that surrounds the tubule and the lumen (L) of the tubule.
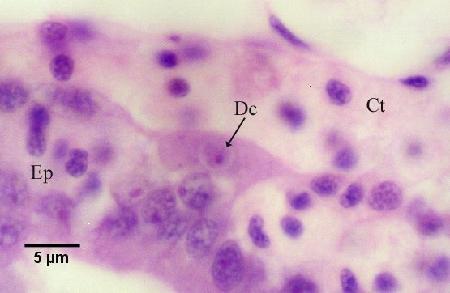
Figure 11. Nurse cell containing one daughter cell (Dc) and residing along the basal membrane of the tubule between the connective tissue (Ct) surrounding the tubules and the tubule epithelium (Ep).
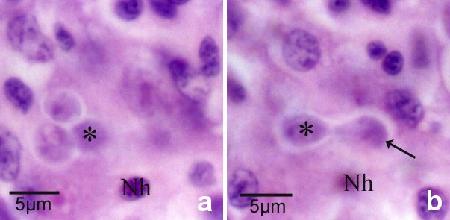
Figure 12a and b. The same tissue section but at different focal planes demonstrates the budding of a daughter cell (arrow in Fig. 12b) within the nurse cell. An asterisk marks the same daughter cell and Nh denotes the same host cell nucleus in each figure. There are two additional daughter cells within the nurse cell (Fig. 12a).
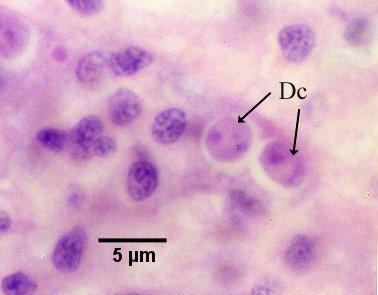
Figure 13. Nurse cell containing two daughter cells (Dc, see phase 5 in the life cycle diagram).
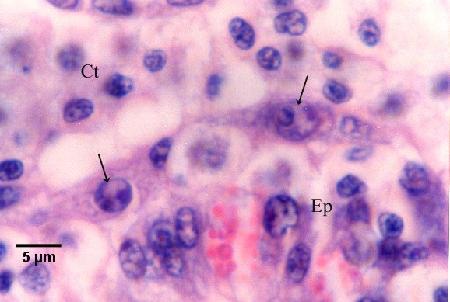
Figure 14. Nurse cells containing bicellular daughter cells (arrows) along the basal membrane between the tubule epithelium (Ep) and the connective tissue (Ct) that contains many infiltrating haemocytes (see phase 6 in the life cycle diagram).
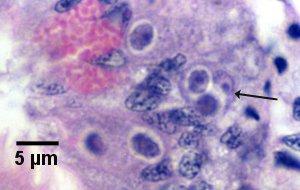
Figure 15. Primary cell (arrow) containing two secondary cells (sporont primordia) just prior to sporulation (see initiation of phase 7 in the life cycle diagram).
Figures 16 and 17. Tissue section through the digestive gland of Saccostrea glomerata demonstrating an advanced stage of disease with sporulating stages of Marteilia sydneyi. Haematoxylin and eosin stain. Images provided by S.N. Kleeman, Biosecurity Australia.
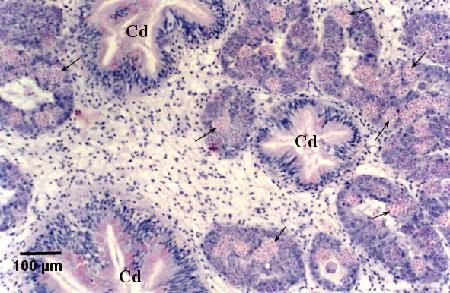
Figure 16. Numerous sporulating stages (arrows) in the digestive gland tubules. Note that sporulation does not occur in the ciliated ducts (Cd) of the digestive gland.
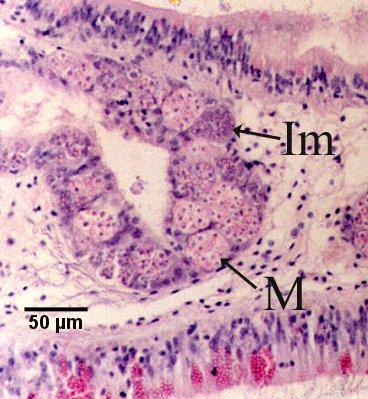
Figure 17. Immature sporonts (Im) and mature sporonts (M) within sporangiosori in a digestive gland tubule. Note that the epithelium of the tubule is almost completely replaced by M. sydneyi.
Electron Microscopy
Formalin fixed material processed for transmission electron microscopy can be used for species determination of M. sydneyi by visualizing the two tri-cellular spores in a sporont (Adlard and Wesche 2005).
Immunological Assay
An Indirect Fluorescent Antibody Test (IFAT) for detecting most stages of M. sydneyi was developed by Roubal and Lester (1987). However, this assay did not react to the contents of the spore (Roubal et al. 1989). Anderson et al. (1994b) determined that the IFAT based on the polyclonal anti-QX antibody developed by Roubal et al. (1989) recognised sporulating stages of M. sydneyi but not those of related species. However, this polyclonal antibody failed to detect presumed presporulation stages of M. sydneyi in the connective tissue of recently infected oysters. Therefore, Anderson et al. (1994b) suggested that antigens of M. sydneyi were stage specific and indicated that a DNA probe rather than immunohistochemical tests may be more useful in detecting this parasite.
DNA Probes
Sequence data for the first internal transcribed spacer (ITS1) region of M. sydneyi rDNA described by Anderson et al. (1995) were used in the development of Polymerase Chain Reaction (PCR) and in situ hybridisation assays (Kleeman and Adlard 2000). PCR assays on known numbers of sporonts purified from the digestive gland of infected oysters indicated that DNA equivalent to 0.01 sporonts was detectable using agarose gel electrophoresis. However, concentrations of host DNA in excess of 50 ng per 20 µl reaction reduced the sensitivity of the test. A DIG-labelled DNA probe constructed from M. sydneyi unique primers could detect 10 pg of M. sydneyi PCR amplified DNA in dot-blot hybridisation. This probe hybridised to all developmental stages of M. sydneyi in paraffin sections of infected oyster digestive gland with no non-specific binding, however, inconsistent detection of mature sporont stages was observed (Kleeman and Adlard 2000). PCR protocols using this probe for the detection of M. sydneyi in oyster tissue were optimised and validated by Adlard and Worthington Wilmer (2003) and reported by Adlard and Wesche (2005). PCR was found to be more sensitive in detecting infection than examining tissue imprints (98.99% infected versus 58.11% in a sample of 1839 oysters) because PCR is able to detect M. sydneyi infections at a much earlier stage of development long before pre-sporulating and sporulating stages are easily identifiable through microscopy in the oyster digestive gland (Adlard and Wesche 2005). However, according to Simonian et al. (2009a), sporulation is a more reliable marker of QX disease than PCR because sporulation corresponds with the disease state, rather than simply reflecting the presence of M. sydneyi. Simonian et al. (2009a) used proteome maps produced by two dimensional electrophoresis to identify significant differences in the expression of four proteins in oysters with sporulating M. sydneyi infections.
The "Smart 2 probe" designed by Le Roux et al. (1999) from within the 18S rRNA of M. refringens also detected M. sydneyi via in situ hybridisation, and, although not a species specific test, allowed more reliable detection of all life cycle stages (Kleeman et al. 2002b). The combined use of the M. sydneyi specific ITS1 probe and the Smart 2 probe in in situ hybridisation allowed elucidation of the occurrence of nurse cells of M. sydneyi between epithelial cells and adjacent to the basal membrane of the digestive gland tubules (Fig. 10). Nurse cells are usually not evident in normal histology stained with haematoxylin and eosin stain. The application of the in situ hybridisation assay to lightly infected oysters allowed the visualisation of early developmental stages of infection (Fig. 9) and determination of a hypothetical development cycle of M. sydneyi in S. glomerata (see Fig. 1 and Kleeman et al. 2002a).
The ITS1 PCR and in situ hybridisation assays proved specific to M. sydneyi when tested for their potential to cross-react with related species of Paramyxea, including Marteilia refringens in Ostrea edulis from France, Marteilioides chungmuensis in Crassostrea gigas from Japan, and Marteilioides sp. from Saccostrea echinata (=Striostrea mytiloides) the blacklip oyster from Darwin Harbour, Australia (Kleeman et al. 2002b). Although the Smart 2 probe (Le Roux et al. 1999) cross-reacted with various species of Paramyxea (Kleeman et al. 2002b), this probe provided a stronger signal in the detection of sporont stages and was more reliable in the detection of mature spores of M. sydneyi than the ITS1 probe (compare Figs. 20 to 19 and Figs. 21 to 22). Thus, Kleeman et al. (2002b) indicated that the Smart 2 probe was preferred for use in the screening or surveillance of oyster populations and that the ITS1 probe should be used as one means of confirming the specific identity of the pathogen as M. sydneyi. Kleeman et al. (2002b) also determined that in the absence of comparative sequence data, primers designed within the internal transcribed spacer regions (ITS) are best suited for the production of species specific PCR tests. The ITS1 and Smart 2 probes were used to detect a possible alternative host of M. sydneyi (Adlard and Nolan 2015).
Figures 18 to 20. Detection of Marteilia sydneyi in the digestive tubules of Saccostrea glomerata. Images provided by S.N. Kleeman, Biosecurity Australia.
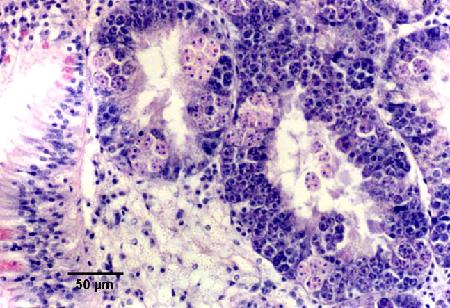
Figure 18. Detection by conventional histology. The epithelium of the digestive gland tubules are almost completely replaced by sporulating stages of M. sydneyi.
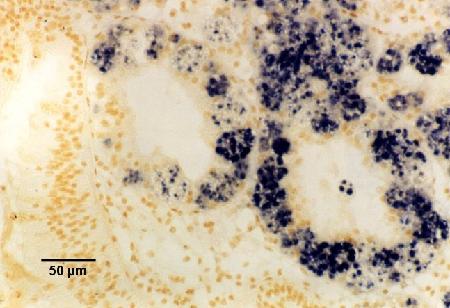
Figure 19. Detection by the ITS1 probe.
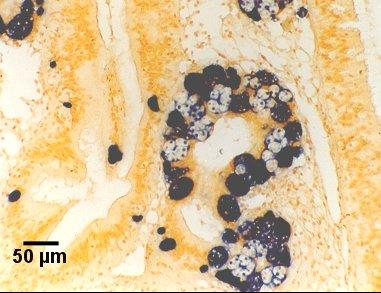
Figure 20. Detection by the Smart 2 probe.
Figures 21 and 22. Detection of immature and mature sporont stages within sporangiosori of Marteilia sydneyi in tissue sections of Saccostrea glomerata by in situ hybridisation. Images provided by S.N. Kleeman, Biosecurity Australia.
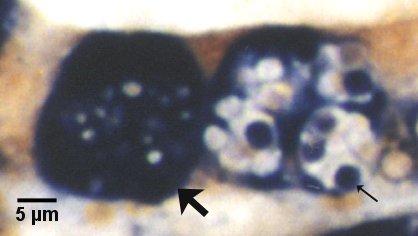
Figure 21. Detection with the Smart 2 probe. Both sporangiosori, one containing immature sporonts (large arrow) and the other with mature sporonts (small arrow) are darkly stained by this probe. Note that the refringent granules (most evident in the sporangiosorus with mature sporonts) are not stained.
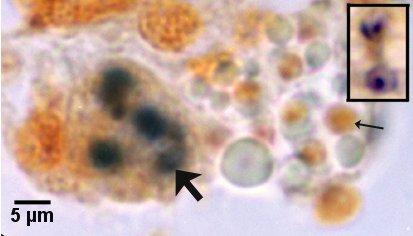
Figure 22. Detection with the ITS1 probe. The sporangiosorus containing immature sporonts (large arrow) is less hybridised than in Fig. 21. Note that most mature spores (small arrow) failed to hybridise. Inset at same magnification illustrates two mature spores that were successfully labeled with the ITS1 probe.
Kleeman et al. (2004) determined that single strand conformation polymorphism (SSCP)-based analysis of a region (195 bp) of the ITS1 of ribosomal DNA could be employed to detect the existence of at least two genetic variant of M. sydneyi. This molecular data supported the proposal of the origin and subsequent southward distribution of this parasite along the south eastern coast of Australia (Kleeman et al. 2004). The conserved rDNA 18S-coding region has been used to demonstrate the phylogenetic relationships among several species of Paramyxida including Marteilia refringens and Marteilia cochillia (Europe), M. sydneyi (Australia), and the Asian species, Marteilia granula (Japan) and Marteilioides chungmuensis (Korea, Japan) (Itoh et al. 2014, Carrasco et al. 2015, Ward et al. 2016).
Methods of control
Disease control is attempted by altering culture techniques: oysters are not planted in areas of risk during the austral summer (January to March), young oysters are held in high salinity water where they grow more slowly but remain free of infection until after the risk of infection is past (late April), large oysters are harvested prior to the onset of the transmission period (before late December) and restocking for grow-out from QX free areas in autumn. In addition, determining the risk factors that result in QX disease outbreaks will allow farmers to position their farms in locations where QX disease outbreaks are less likely to occur (Green et al. 2011). For example, hanging clumps of oysters from flotation such as from rafts or barrels (Wisely et al. 1979) or grow-out of oysters at higher levels in the intertidal zone in enzootic areas (Wolf 1979, Meyer 2006) may reduce exposure of the farmed oysters to M. sydneyi. Farm management practices of transferring oyster stocks between estuaries increases the risk of introducing the parasite to new areas (Guo and Ford 2016). Legislation (disease zoning) in New South Wales has been in force since 1986 to prevent the transfer of potentially infected stocks from QX-endemic areas to QX-free southern estuaries (Nell 1993, 2001; Adlard and Ernst 1995). However, this control measure hindered the farming of S. glomerata which historically relied on transferring wild-caught spat between estuaries for on-growing to market size and did not prevent the occurrence of QX disease in new locations (Green et al. 2011). Although the legislation approach was chosen purely because it was the only management tool available at that time to prevent QX-disease, the rationale for its implementation was flawed because QX disease occurred in only a minority of NSW estuaries, but the disease agent, M. sydneyi, was present in the majority of NSW estuaries (Adlard and Wesche 2005, Carrasco et al. 2015). Adlard and Wesche (2005) concluded that QX disease was caused either by an environmental trigger, an abundance of the intermediate host in the environment, and/or immunosuppression of the host. Different strains of M. sydneyi also exist in the river systems of Southern Queensland and Northern NSW (Kleeman et al. 2004), but it is not known if they differ in their pathogenicity. If the different strains do vary in pathogenicity, it may be appropriate to design QX-disease risk based closures around limiting the spread of different M. sydneyi variants between river systems (Carrasco et al. 2015). According to Green et al. (2011) the future of the S. glomerata industry relies in part on the successful commercialization of hatchery-produced QX-disease resistant S. glomerata.
To control the disease, a QX disease resistance-breeding program was established in 1996 by the Australian New South Wales Department of Primary Industries (Nell et al. 2000, Nell 2001, Nell and Hand 2003, Dove et al. 2013a). Initially, it was reported that phenoloxidase activity was enhanced in S. glomerata selectively bred for resistance to QX disease (Newton et al. 2004). Unfortunately, about 10 years (four generations) of selective breeding of these oysters inadvertently resulted in a stock with significantly less phenoloxidase POb a defensive enzyme associated with resistance to disease (Bezemer et al. 2006). Nevertheless, Nell and Perkins (2006) and Dove et al. (2013a, b) reported that progeny of third- and fourth-generation, respectively, S. glomerata selected breeding lines had evaluated resistance to disease caused by both M. sydneyi and Bonamia roughleyi compared to non-selected controls. But, selection for resistance to M. sydneyi did not appear to confer resistance to B. roughleyi and the converse also applied (Nell and Perkins 2006; Dove et al. 2013a, b). However, the selection process that was employed may have allowed for the selection of broodstock that had been challenged by multiple diseases and thus acquired some resistance to some of them (Green et al. 2008, 2009). Simonian et al. (2009b) developed a proteomic approach using 2-dimensional electrophoresis and mass spectrometry to identify two potential markers of QX disease resistance that were in addition to phenoloxidase activity. Kuchel et al. (2010) suggested that resistance against QX disease may be associated with enhanced phagolysosomal activity in QX disease resistant oysters. Dang et al. (2011) analysed the haemocytic response of S. glomerata that were progeny of the fifth generation of oysters bred for QX resistance in comparison to non-selected S. glomerata using flow cytometry and histology for assessing parasite viability. They determined that amongst the QX resistant oysters, 20% were diseased containing viable parasite, 74% had killed M. sydneyi and 6% were uninfected. In contrast, 86% of non-selected oysters were diseased, 2% had killed M. sydneyi and 12% were healthy. Although the rate of phagocytosis and the mean oxidative activity per haemocyte were similar between both oyster lines, the QX resistant oysters had higher numbers of infiltrating and circulating haemocytes, and a higher percentage of circulating granulocytes, which were larger in size and complexity than those of non-selected oysters, High abundance of M. sydneyi in the digestive tubule epithelium of both oyster lines implied inability to kill the parasite at the beginning of the infection. However, QX resistant oysters were able to kill the sporangiosorus of M. sydneyi, probably by the process of encapsulation (Dang et al. 2011).
Jenkins et al. (2013) indicated that Crassostrea gigas (not native to Australia) has become the major commercial oyster in Tasmania and South Australia and is preferentially farmed in some New South Wales (NSW) estuaries due to the impact of QX disease (caused by M. sydneyi) on the indigenous species S. glomerata. In the Georges River, NSW, triploid C. gigas are farmed alongside S. glomerata, where it generally takes approximately 7 to 18 months to grow small spat (shell height: 4 mm) of C. gigas to a marketable size (shell height: 50 to 120 mm). However, in the Parramatta River (Sydney Harbour), only wild populations of diploid C. gigas and S. glomerata are present; with C. gigas considered an invasive pest species. In both these areas, high mortalities (greater than 95%) of C. gigas caused by ostreid herpesvirus-1 (OsHV-1) started in the summer (November) 2010. Fortunately, nearby stocks of S. glomerata were not affected and S. glomerata seems resistant to infection by OsHV-1 (Jenkins et al. 2013).
Wesche et al. (1999) determined that spores of M. sydneyi were relatively short-lived once isolated from oysters and that the majority die within 7 to 9 days (maximum longevity was 35 days at 15° C and 34 ppt salinity). Spores did not survive more than 2 hours following ingestion by birds or fish but they remained viable for over 7 months at -20 and -70° C. Exposure to chlorine (from granular calcium hypochlorite 650 g per kg) at 200 ppm killed 99.5% of the spores within 2 hours and all spores within 4 hours of exposure (Wesche et al. 1999).
References
- Adlard, R.D. 1997. QX disease (Marteilia sydneyi) of the Sydney rock oyster (Saccostrea commercialis) on the central coast of NSW. Final report for Fisheries Research and Development Corporation, FRDC Project No. 1994/156. The University of Queensland, Brisban, Australia.
- Adlard, R.D. and I. Ernst. 1995. Extended range of the oyster pathogen Marteilia sydneyi. Bulletin of the European Association of Fish Pathologists 15: 119-121.
- Adlard, R.D. and M.J. Nolan. 2009. Identification of host interactions in the life cycle of QX disease, Final Report for Fisheries Research and Development Corporation, Aquatic Animal Health Subprogram FRDC Project No. 2006/062. Queensland Museum, Brisbane, Australia.
- Adlard, R.D. and M.J. Nolan. 2015. Elucidating the life cycle of Marteilia sydneyi, the aetiological agent of QX disease in the Sydney rock oyster (Saccostrea glomerata). International Journal for Parasitology 45: 419-426.
- Adlard, R.D. and S.J. Wesche. 2005. Aquatic Animal Health Subprogram: Development of a disease zoning policy for Marteilia sydneyi to support sustainable production, health certification and trade in the Sydney rock oyster. Final Report 2001/214. Australian Fisheries Research and Development Corporation and Queensland Museum, Brisbane, Australia, pp. 1-46.
- Adlard, R. and J. Worthington-Wilmer. 2003. Validation of DNA-based (PCR) diagnostic tests suitable for use in surveillance programs for QX disease of Sydney rock oysters (Saccostrea glomerata) in Australia. Final report for Fisheries Research and Development Corporation, Aquatic Animal Health Subprogram FRDC Project No. 2001/630. Queensland Museum, Brisban, Australia.
- Anderson, I.G. 1990. Diseases in Australian invertebrate aquaculture. In: Proceedings and Abstracts, Fifth International Colloquium on Invertebrate Pathology and Microbial Control, Society for Invertebrate Pathology, 20-24 Aug. 1990, Adelaide, Australia, p. 38-48.
- Anderson, T.J., S. Wesche and R.J.G. Lester. 1994a. Are outbreaks of Marteilia sydneyi in Sydney rock oysters, Saccostrea commercialis, triggered by a drop in environmental pH? Australian Journal of Marine and Freshwater Research 45: 1285-1287.
- Anderson, T.J., T.F. McCaul, V. Boulo, J.A.F. Robledo and R.J.G. Lester. 1994b. Light and electron immunohistochemical assays on paramyxea parasites. Aquatic Living Resources 7: 47-52.
- Anderson, T.J., R.D. Adlard and R.J.G. Lester. 1995. Molecular diagnosis of Marteilia sydneyi (Paramyxea) in Sydney rock oysters, Saccostrea commercialis (Angas). Journal of Fish Diseases 18: 507-510.
- Aranguren, R. and A. Figueras. 2016. Moving from histopathology to molecular tools in the diagnosis of molluscs diseases of concern under EU Legislation. Frontiers in Physiology 7: Article 538, 10 pp.
- Berthe, F.C.J., F. Le Roux, E. Peyretaillade, P. Peyret, D. Rodriguez, M. Gouy and C.P. Vivarès. 2000. Phylogenetic analysis of the small subunit ribosomal RNA of Marteilia refringens validates the existence of Phylum Paramyxea (Desportes and Perkins 1990). The Journal of Eukaryotic Microbiology 47: 288-293.
- Berthe, F.C.J., F. Le Roux, R.D. Adlard and A. Figueras. 2004. Marteiliosis in molluscs: A review. Aquatic Living Resources 17: 433-448.
- Bezemer, B., D. Butt, J. Nell, R. Adlard and D. Raftos. 2006. Breeding for QX disease resistance negatively selects one form of the defensive enzyme, phenoloxidase, in Sydney rock oysters. Fish and Shellfish Immunology 20: 627-636.
- Butt, D. and D. Raftos. 2007. Immunosuppression in Sydney rock oysters (Saccostrea glomerata) and QX disease in the Hawkesbury River, Sydney. Marine and Freshwater Research 58: 213-221.
- Butt, D. and D. Raftos. 2008. Phenoloxidase-associated cellular defence in the Sydney rock oyster, Saccostrea glomerata, provides resistance against QX disease infections. Developmental and Comparative Immunology 32: 299-306.
- Carrasco, N., T.J. Green and N. Itoh. 2015. Marteilia spp. parasites in bivalves: A revision of recent studies. Journal of Invertebrate Pathology 131: 43-57.
- Cavalier-Smith, T. and E.E.Y. Chao. 2003. Phylogeny and Classification of Phylum Cercozoa (Protozoa). Protist 154: 341-358.
- Comps, M. 1976. Marteilia lengehi n. sp., parasite de l'huître Crassostrea cucullata Born. Revue des Travaux de l'Institut des Pêches Maritimes 40: 347-349. (In French with English Summary).
- Dang, C., C. Lambert, P. Soudant, J. Delamare-Deboutteville, M.M. Zhang, J. Chan, T.J. Green, N. Le Goïc and A.C. Barnes. 2011. Immune parameters of QX-resistant and wild caught Saccostrea glomerata hemocytes in relation to Marteilia sydneyi infection. Fish & Shellfish Immunology 31: 1034-1040.
- Diggles, B.K. 2013. Historical epidemiology indicates water quality decline drives loss of oyster (Saccostrea glomerata) reefs in Moreton Bay, Australia. New Zealand Journal of Marine and Freshwater Research 47: 561-581.
- Dove, M.C., J.A. Nell, S. Mcorrie and W.A. O'Connor. 2013a. Assessment of Qx and winter mortality disease resistance of mass selected Sydney rock oysters, Saccostrea glomerata (Gould 1850), in the Hawkesbury River and Merimbula Lake, NSW Australia. Journal of Shellfish Research 32: 681-687.
- Dove, M.C., J.A. Nell and W.A. O'Connor. 2013b. Evaluation of the progeny of the fourth-generation Sydney rock oyster Saccostrea glomerata (Gould 1850) breeding lines for resistance to QX disease (Marteilia sydneyi) and winter mortality (Bonamia roughleyi). Aquaculture Research 44: 1791-1800.
- Feist, S.W., P.M. Hine, K.S. Bateman, G.D. Stentiford and M. Longshaw. 2009. Paramarteilia canceri sp. n. (Cercozoa) in the European edible crab (Cancer pagurus) with a proposal for the revision of the order Paramyxida Chatton 1911. Folia Parasitologica 56: 73-85.
- Green, T.J. and A.C. Barnes. 2010. Bacterial diversity of the digestive gland of Sydney rock oysters, Saccostrea glomerata infected with the paramyxean parasite, Marteilia sydneyi. Journal of Applied Microbiology 109: 613-622.
- Green, T.J., B.J. Jones, R.D. Adlard and A.C. Barnes. 2008. Parasites, pathological conditions and mortality in QX-resistant and wild-caught Sydney rock oysters, Saccostrea glomerata. Aquaculture 280: 35–38.
- Green, T.J., T.J. Dixon, E. Devic, R.D. Adlard and A.C. Barnes. 2009. Differential expression of genes encoding anti-oxidant enzymes in Sydney rock oysters, Saccostrea glomerata (Gould) selected for disease resistance. Fish & Shellfish Immunology 26: 799-810.
- Green, T.J., D. Raftos, W. O'Connor, R.D. Adlard and A.C. Barnes. 2011. Disease prevention strategies for QX disease (Marteilia sydenyi) of Sydney rock oysters (Saccostrea glomerata). Journal of Shellfish Research 30: 47-53.
- Guo, X. and S.E. Ford. 2016. Infectious diseases of marine molluscs and host responses as revealed by genomic tools. Philosophical Transactions of the Royal Society B: Biological Sciences 371: 20150206, 16pp.
- Hine, P.M. 1996. Southern hemisphere mollusc diseases and an overview of associated risk assessment problems. Revue Scientifique et Technique de l'Office International des Epizooties 15: 563-577.
- Hine, P.M. and T. Thorne. 2000. A survey of some parasites and diseases of several species of bivalve mollusc in northern Western Australia. Diseases of Aquatic Organisms 40: 67-78.
- Itoh, N., T. Yamamoto, H.-S. Kang, K.-S. Choi, T.J. Green, N. Carrasco, M. Awaji and S. Chow. 2014. A novel paramyxean parasite, Marteilia granula sp. nov. (Cercozoa), from the digestive gland of Manila clam Ruditapes philippinarum in Japan. Fish Pathology 49: 181-193.
- Jenkins, C., P. Hick, M. Gabor, Z. Spiers, S.A. Fell, X. Gu, A. Read, J. Go, M. Dove, W. O'Connor, P.D. Kirkland and J. Frances. 2013. Identification and characterisation of an ostreid herpesvirus-1 microvariant (OsHV-1 µ-var) in Crassostrea gigas (Pacific oysters) in Australia. Diseases of Aquatic Organisms 105: 109-126.
- Jones, J.B. and J. Creeper. 2006. Diseases of pearl oysters and other molluscs: a Western Australian perspective. Journal of Shellfish Research 25: 233-238.
- King, W.L., C. Jenkins, J.R. Seymour and M. Labbate. 2019. Oyster disease in a changing environment: Decrypting the link between pathogen, microbiome and environment. Marine Environmental Research 143: 124-140.
- Kleeman, S.N. and R.D. Adlard. 2000. Molecular detection of Marteilia sydneyi, pathogen of Sydney rock oysters. Diseases of Aquatic Organisms 40: 137-146.
- Kleeman, S.N., R.D. Adlard and R.J.G. Lester. 2001. Detection of early infective stages of Marteilia sydneyi and their development through to sporogenesis. Book of Abstracts, European Association of Fish Pathologists, Tenth International Conference "Diseases of Fish and Shellfish". Trinity College Dublin, Ireland, 9 - 14 September 2001. pg. O-004.
- Kleeman, S.N., R.D. Adlard and R.J.G. Lester. 2002a. Detection of the initial infective stages of the protozoan parasite Marteilia sydneyi in Saccostrea glomerata and their development through to sporogenesis. International Journal for Parasitology 32: 767-784.
- Kleeman, S.N., F. Le Roux, F. Berthe and R.D. Adlard. 2002b. Specificity of PCR and in situ hybridization assays designed for detection of Marteilia sydneyi and M. refringens. Parasitology 125: 131-141.
- Kleeman, S., R. Adlard and B. Lester. 2002c. Discovery of the early infective stage of the protozoan parasite Marteilia sydneyi in oysters and the implications for disease detection and control. Handbook and Abstracts, Fifth Symposium on Diseases in Asian Aquaculture, Queensland, Australia, 24-28 November 2002. Pg. 100.
- Kleeman, S.N., R.D. Adlard, X. Zhu and R.B. Gasser. 2004. Mutation scanning analysis of Marteilia sydneyi populations from different geographical locations in eastern Australia. Molecular and Cellular Probes 18: 133-138.
- Kuchel, R.P., S. Aladaileh, D. Birch, N. Vella and D.A. Raftos. 2010. Phagocytosis of the protozoan parasite, Marteilia sydneyi, by Sydney rock oyster (Saccostrea glomerata) hemocytes. Journal of Invertebrate Pathology 104: 97-104.
- Le Roux, F., C. Audemard, A. Barnaud and F. Berthe. 1999. DNA Probes as potential tools for the detection of Marteilia refringens. Marine Biotechnology 1: 588-597.
- Lester, R.J.G. 1986. Field and laboratory observations on the oyster parasite Marteilia sydneyi. In: M. Cremin, C. Dobson and D.E. Moorhouse (eds), 'Parasite Lives'. University of Queensland Press, pp. 33-40.
- Meyers, T. 2006. 5.2.9 Marteiliasis and marteilioidiasis of shellfish. In: Fish Health Section Blue Book, 2016 Edition, Suggested Procedures for the Detection and Identification of Certain Finfish and Shellfish Pathogens. American Fisheries Society's Fish Health Section.
- Nell, J.A. 1993. Farming the Sydney rock oyster (Saccostrea commercialis) in Australia. Reviews in Fisheries Science 1: 97-120.
- Nell, J.A. 2001. The history of oyster farming in Australia. Marine Fisheries Review 63: 14-25.
- Nell, J. 2002. The Australian oyster industry. World Aquaculture 33: 8-10.
- Nell, J.A. and R.E. Hand. 2003. Evaluation of the progeny of second-generation Sydney rock oyster Saccostrea glomerata (Gould 1850) breeding lines for resistance to QX disease Marteilia sydneyi. Aquaculture 228: 27-35.
- Nell, J.A. and B. Perkins. 2006. Evaluation of the progeny of third-generation Sydney rock oyster Saccostrea glomerata (Gould 1850) breeding lines for resistance to QX disease Marteilia sydneyi and winter mortality Bonamia roughleyi. Aquaculture Research 37: 693-700.
- Nell, J.A., I.R. Smith and C.C. McPhee. 2000. The Sydney rock oyster Saccostrea glomerata (Gould 1850) breeding program: progress and goals. Aquaculture Research 31: 45-49.
- Newton, K., R. Peters and D. Raftos. 2004. Phenoloxidase and QX disease resistance in Sydney rock oysters (Saccostrea glomerata). Developmental and Comparative Immunology 28: 565-569.
- Perkins, F.O. and P.H. Wolf. 1976. Fine structure of Marteilia sydneyi sp. n. - haplosporidan pathogen of Australian oysters. The Journal of Parasitology 62: 528-538.
- Peters, R. and D.A. Raftos. 2003. The role of phenoloxidase suppression in QX disease outbreaks among Sydney rock oysters (Saccostrea glomerata). Aquaculture 223: 29-39.
- Potter, M.A. 1983. Growth rates of cultivated Sydney rock oysters, Saccostrea (Crassostrea) commercialis, in two estuaries in subtropical southern Queensland. Queensland Journal of Agriculture and Animal Science 40: 137-140.
- Roubal, F.R., J. Masel and R.J.G. Lester. 1989. Studies on Marteilia sydneyi, agent of QX disease in the Sydney rock oyster, Saccostrea commercialis, with implications for its life cycle. Australian Journal of Marine and Freshwater Research 40: 155-167.
- Rubio, A., J. Frances, P. Coad, J. Stubbs and K. Guise. 2013. The onset and termination of the QX Disease window of infection in Sydney rock oyster (Saccostrea glomerata) cultivated in the Hawkesbury River, NSW, Australia. Journal of Shellfish Research 32: 483-496.
- Simonian, M., S.V. Nair, W.A. O'Connor and D.A. Raftos. 2009a. Protein markers of Marteilia sydneyi infection in Sydney rock oysters, Saccostrea glomerata. Journal of Fish Diseases 32: 367-375.
- Simonian, M., S.V. Nair, J.A. Nell and D.A. Raftos. 2009b. Proteomic clues to the identification of QX disease-resistance biomarkers in selectively bred Sydney rock oysters, Saccostrea glomerata. Journal of Proteomics 73: 209-217.
- Taveekijakarn, P., G. Nash, T. Somsiri and S. Putinaowarat. 2002. Marteilia-like species: first report in Thailand. The Aquatic Animal Health Research Institute Newsletter 11: 1-2.
- Taveekijakarn, P., T. Somsiri, S. Puttinaowarat, S. Tundavanitj, S. Chinabut and G. Nash. 2008. Parasitic fauna of rock oyster (Saccostrea forskali) cultured in Thailand. In: Bondad-Reantaso, M.G., Mohan, C.V., Crumlish, M. and Subasinghe, R.P. (eds.) Diseases in Asian Aquaculture VI, proceedings of The Sixth Symposium on Diseases in Asian Aquaculture held in Colombo, Sri Lanka in 2005. Fish Health Section, Asian Fisheries Society, Manila, Philippines, pp. 335-342.
- Ward, G.M., M. Bennett, K. Bateman, G.D. Stentiford, R. Kerr, S.W. Feist, S.T. Williams, C. Berney and D. Bass. 2016. A new phylogeny and environmental DNA insight into paramyxids: an increasingly important but enigmatic clade of protistan parasites of marine invertebrates. International Journal of Parasitology 46: 605-619.
- Wesche, S.J. 1995. Outbreaks of Marteilia sydneyi in Sydney rock oysters and their relationship with environmental pH. Bulletin of the European Association of Fish Pathologists 15: 23-27.
- Wesche, S.J., R.D. Adlard and R.J.G. Lester. 1999. Survival of spores of the oyster pathogen Marteilia sydneyi (Protozoa, Paramyxea) as assessed using fluorogenic dyes. Diseases of Aquatic Organisms 36: 221-226.
- Wisely, B., J.E. Holliday and B.L. Reid. 1979. Experimental deepwater culture of the Sydney rock oyster (Crassostrea commercialis = Saccostrea cucullata). 1. Growth of vertical clumps of oysters ("ren"). Aquaculture 16: 127-140.
- Wolf, P.H. 1972. Occurrence of a haplosporidan in Sydney rock oysters Crassostrea commercialis from Moreton Bay, Queensland, Australia. Journal of Invertebrate Pathology 19: 416-417.
- Wolf, P.H. 1977. Diseases and parasites in Australian commercial shellfish. Haliotis 8: 75-83.
- Wolf, P.H. 1979. Life cycle and ecology of Marteilia sydneyi in the Australian oyster, Crassostrea commercialis. Marine Fisheries Review 41 (1-2): 70-72.
Citation information
Bower, S.M. (2019): Synopsis of Infectious Diseases and Parasites of Commercially Exploited Shellfish: Marteilia sydneyi of Oysters
Date last revised: November 2020
Comments to Susan Bower
- Date modified: